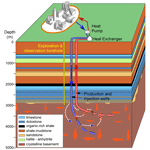
Borehole research in New York State can advance utilization of low-enthalpy geothermal energy, management of potential risks, and understanding of deep sedimentary and crystalline geologic systems
Patrick Fulton
Jefferson Tester
David Bruhn
Hiroshi Asanuma
Ulrich Harms
Chaoyi Wang
Doug Schmitt
Philip J. Vardon
Hannes Hofmann
Tom Pasquini
Jared Smith
In January 2020, a scientific borehole planning workshop sponsored by the International Continental Scientific Drilling Program was convened at Cornell University in the northeastern United States. Cornell is planning to drill test wells to evaluate the potential to use geothermal heat from depths in the range of 2700–4500 m and rock temperatures of about 60 to 120 ∘C to heat its campus buildings. Cornell encourages the Earth sciences community to envision how these boreholes can also be used to advance high-priority subsurface research questions. Because nearly all scientific boreholes on the continents are targeted to examine iconic situations, there are large gaps in understanding of the “average” intraplate continental crust. Hence, there is uncommon and widely applicable value to boring and investigating a “boring” location. The workshop focused on designing projects to investigate the coupled thermal–chemical–hydrological–mechanical workings of continental crust. Connecting the practical and scientific goals of the boreholes are a set of currently unanswered questions that have a common root: the complex relationships among pore pressure, stress, and strain in a heterogeneous and discontinuous rock mass across conditions spanning from natural to human perturbations and short to long timescales. The need for data and subsurface characterization vital for decision-making around the prospective Cornell geothermal system provides opportunities for experimentation, measurement, and sampling that might lead to major advances in the understanding of hydrogeology, intraplate seismicity, and fluid/chemical cycling. Subsurface samples could also enable regional geological studies and geobiology research. Following the workshop, the U.S. Department of Energy awarded funds for a first exploratory borehole, whose proposed design and research plan rely extensively on the ICDP workshop recommendations.
- Article
(4647 KB) - Full-text XML
- BibTeX
- EndNote
Global warming and climate change have become the ultimate challenge for a sustainable environment from now into the foreseeable future. The key to minimizing their impacts is to utilize renewable energy sources to significantly reduce or neutralize the carbon footprint of human activities. Geothermal energy is one widely available resource within the set of available and promising resources which, if intensively deployed, would deliver this environmental value. However, the lack of fundamental scientific and engineering understanding of the subsurface conditions that control the transfer of deeply sourced heat in rock to circulating fluids, along with a need to better understand and manage induced seismicity, has impeded the widespread commercialization of geothermal resources. The pragmatic barrier is that a high investment cost must be paid in advance, while we remain unable to reliably predict that heat can be produced. The potential scientific breakthroughs which can improve predictability of success, costs, and risks would also advance fundamental understanding of continental crust.
More than one-quarter of the world's population lives in the temperate to polar climates north of 35∘ N and south of 35∘ S latitude where, for most of them, the mean annual temperature is less than 11 ∘C (52 ∘F) (Kummu and Varis, 2011). Under those conditions, hot water and space heating are needed for residences and for most indoor working environments. For the 85 % of that high-latitude population who reside in the European Union, United States, and Russia, the consumption of low-temperature heat in homes and commercial buildings is a large fraction (about 21 %) of total energy used (see Sect. 2). A future carbon-neutral world necessitates shifting the source of this heat from combustion of fossil fuels to a renewable, widely available, low-carbon energy source.
A thermal resource available almost everywhere is geothermal energy. However, at present only high-enthalpy volcanic systems and young rift zones are utilized commercially for electric power generation, and low-temperature geothermal resources are underexploited and underexplored. The utilization of 55–85 ∘C water from the Dogger sedimentary rock aquifer near Paris, France, to heat over 200 000 housing units exemplifies the potential (https://www.brgm.eu/project/geothermal-database-on-dogger-aquifer-paris-basin, last access: 20 November 2020). Likewise, geothermal heat from the Delft Sandstone has grown to support 3 % of the heat used for greenhouse horticulture in the Netherlands (https://www.ebn.nl/wp-content/uploads/2018/04/EBN-poster-numbers2016.pdf, last access: 20 November 2020). Yet both those cases also exemplify the limitations: our current ability to extract water that carries the geothermal heat is limited to places with high fluid transmissivity, and those optimum rock conditions are not widespread at the subsurface depths with suitable temperatures. From the perspective of routine drilling technology, depth itself is not a problem, although it is an expense, as subsurface temperatures in the range of 50–100 ∘C are obtained at most locations at moderate depths (< 3 km). Rather, the main physical limitations are low porosity and low permeability. Vast areas of the continents lack the natural hydrological capacity traditionally sought in the high-enthalpy systems, as a consequence of the fact that crystalline basement rocks occur either at the surface or within a few kilometers' depth below the surface. The transmissivity of those rocks is likely low and, in general, poorly documented. If this natural geothermal heat resource is to significantly help move human communities off of fossil fuels, what is currently a highly uncertain geothermal technology must advance to become a low-risk, routine option among sources of heat.
One of the typical geological environments with untapped potential at a few kilometers' depth is the lower strata of sedimentary basins and the underlying crystalline basement (Camp et al., 2018; Limberger et al., 2018). The possibility that Cornell University may drill an exploratory borehole to a depth in the range of 4–5 km, which will penetrate nearly 3 km of Paleozoic sedimentary rocks and ≥ 1 km of granulite-grade Grenville metamorphic rocks, sets up an opportunity for novel scientific experimentation to examine the stress state, hydrogeological and geomechanical conditions, and the rock and fluid responses to perturbations, both natural and anthropogenic. Using such a borehole laboratory, there will be broad applicability for the understanding to be gained of the state of stress within crystalline basement in an area of very low natural seismicity and of how low-permeability sedimentary rocks as well as heterogeneous mid- to high-grade metamorphic rocks near the sedimentary–basement contact respond to the complex relationships between pore pressure, stress and strain.
In an idealized geothermal energy extraction project, heated fluids are extracted, the energy is harvested, the cooled fluids are recycled by injection into a target formation to absorb again the geothermal heat, and the fluid and heat cycling continues. Yet near Cornell University and many other places globally, natural in situ conditions are not likely to provide sufficient contact of circulating fluids with rock surfaces to achieve acceptable rates of geothermal energy extraction. In such cases, enhanced geothermal system (EGS) technologies are needed to promote fluid circulation and fluid–rock contact area. However, drilling, artificial permeability improvement, and fluid cycling all cause perturbations in the subsurface that may have hazardous consequences, ranging from induced seismicity to contamination of groundwater with geothermal fluids. The EGS activities may also fail to achieve the energy extraction targets, which creates very high financial risks for an EGS.
Interestingly, the uncertainties of induced seismic risk and of financial risk are rooted in the same scientific unknowns: the complex relationships among pore pressure, stress, and strain in rock with pre-existing heterogeneity, across conditions spanning from natural to short-duration human perturbation (e.g., stimulation of fractures) and to long-term human forcings (e.g., sustained injection and production of water supplies, hydrocarbons, or geothermal brines).
Knowledge of geological features, temperature profile, stress variations, and permeability with increasing depth and with changing rock type is essential to ensure the proper deployment of EGS applications while minimizing the environmental impact. However, in continental crust, a surprising lack of understanding of stress and permeability hinders practical developments of EGS at the scale necessary for real applications in which benefits balance costs. Our primary scientific goal is to gain an understanding of the spatial distribution and temporal evolution of stress and permeability with depth and the intertwined relationships with temperature, lithology, faults, natural fracture groups, rock fabric, and mineral dissolution and precipitation. This knowledge will elevate the understanding of (1) how the near-field crust immediately reacts to changes in pore pressure, geochemistry, and resultant thermo-chemo-poroelastic effects; (2) the influences on far-field crust accounting for a stress halo and fluid migration; and (3) the risks of induced seismicity and contamination.
Consequently, a borehole needed to provide data vital for making decisions about the prospective Cornell geothermal system can also be an opportunity for experimentation, measurement, and sampling that might lead to major scientific advances in the understanding of crustal hydrology, earthquakes in plate interiors, and fluid-chemical cycling. To explore the merits of turning a Cornell test borehole into a broader borehole of scientific opportunity, the ICDP sponsored a borehole science planning workshop at Cornell University on 8–10 January 2020.
Central New York State in northeastern North America is just one location, yet its need for the conversion to a low-carbon energy source by which to warm residences and commercial buildings and to heat water is an extremely common situation for much of Earth's population. Cornell University seeks to lead the way in demonstrating one path forward that could be widely deployed.
2.1 Carbon neutrality in temperate climate, cloudy, heavily populated areas is not easy
Cornell University selected 2035 as the date by which to become carbon-neutral on its Ithaca, New York, campus. The goal was adopted without an operational plan that was feasible using available technologies of the adoption year, 2013. A similar pledge was made in 2019 by the State of New York in the cold-climate northeastern USA on stable North American continental crust. The state pledged to eliminate net greenhouse gas emissions by 2050, also without an existing plan encompassing the removal of carbon from the energy used to heat residential and commercial buildings. In New York State, the contribution of heating to carbon emissions is large: about 35 % of New York's annual primary energy is consumed for heating, using energy sources dominated by the combustion of natural gas, fuel oil and propane. In New York State, on-site fossil fuel combustion in the residential, commercial and industrial sectors – all of which predominantly supply low-temperature space and water heating – contributes 30 % of greenhouse gas emissions, compared to electricity production contributing 13 % and transportation 36 % (EIA, 2018a, b, 2019; McCabe et al., 2016; NYSERDA, 2019). The comparatively low carbon footprint of New York's electricity generation is due to major hydropower and nuclear contributions. It is evident that the eventual successful decarbonization of the heating of homes and commercial buildings in heavily populated regions demands an overhaul of the energy supply for heating, such as by use of geothermal energy.
The concept under evaluation by Cornell University, to utilize geothermal heat by tapping fluids in rock at temperatures in the range of 60–120 ∘C, could also serve widely in New York State to heat residences and commercial buildings, for certain industrial activities, and to assist controlled agriculture operations for food production. Although New York State has banned high-volume hydraulic fracturing in horizontal wells, as practiced for production of gas or oil from organic-rich shale, the long-established use of hydraulic pressure to stimulate existing fractures remains legal. Hence both the options of natural transmissivity and fluid flow through stimulated fractures are plausible fluid-flow pathways. Nevertheless, the technical uncertainty and investment risks for direct-use geothermal energy projects are dominated by the uncertainty about establishment of fluid flow through a well field that is sufficiently dispersed to sweep heat from rock surfaces in a large volume of rock, rather than to short-circuit through a small set of flow paths. The university's view of the next step to test the opportunity is that there are three intertwined objectives: to develop a demonstration reservoir using a well pair that will deliver 20 % of Cornell's heating demand, to de-risk the deployment of geothermal direct-use heating throughout New York and other states with similar geology and climate, and to engender activities that are in keeping with the core university mission: research advances, education, and outreach with novel solutions for broader societal issues.
2.2 Accessing geothermal resources where continental geology is “normal”: a world class problem at a geologically representative site
The ICDP-supported workshop focused on land owned by Cornell University in Ithaca, NY, USA, where the surface geology consists of Devonian sedimentary rock and the subsurface geology includes 2.80 km ± 0.2 km of Paleozoic sedimentary rocks overlying complex Precambrian-age metamorphic rocks (Fig. 1). Nearby, the sedimentary rocks are documented (Camp et al., 2018; Al Aswad, 2019) and the metamorphic rocks inferred to be of very low permeability. According to Limberger et al. (2018), across 16 % of Earth's land surface there exist generally comparable geothermal reservoir systems: a thick sedimentary column over continental basement. That statistic demonstrates the commonality of Ithaca, NY, with a large fraction of the globe. Nevertheless, for central New York, the paucity of sufficient porosity in all but a tiny fraction of the strata sets the possible geothermal reservoirs apart from the desirable “Hot Sedimentary Aquifers” reservoir category considered to be especially good targets for geothermal heat extraction (Wright and Culver, 1991), like the highly permeable Dogger Limestone of the Paris Basin (Le Brun et al., 2011). Instead, the central New York State data lead to anticipation that the conditions will be more like those documented by Dillinger et al. (2016) in the Cooper Basin of Australia, where complex diagenesis resulted in low permeability and lack of sufficient fluid production at a geothermal test well. For Cornell, it is likely that access to the geothermal heat will require fracture flow, which puts in the spotlight the natural characteristics of fractures in both sedimentary and metamorphic rocks and raises key questions about the behavior of those fractures in the ambient stress field under the perturbed conditions that might be imposed in an effort to hydraulically stimulate flow along fractures or that might develop during long-term geothermal field production.
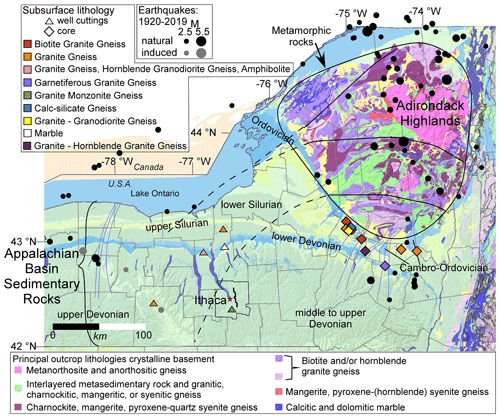
Figure 1Map of geology and historical earthquakes in New York State. Geological map shows bedrock composition immediately subjacent to soil and Holocene sediments (USGS map source, https://mrdata.usgs.gov/geology/state/map.html?x=-75.6897884252848&y=42.9882351360762&z=7#, last access: 20 November 2020). The Adirondack Mountains in northern New York are enclosed in a subcircular black polygon. For sedimentary rocks of the Appalachian Basin, the geological periods corresponding to some of the mapped colors are labeled on the map. Subsurface basement lithologies are known from petrographic analysis of samples of cores and cuttings that are archived by the New York State Museum (Benjamin R. Valentino, unpublished data, 2016). Positions of major shear zones of the Adirondack crystalline rocks (solid black lines) are from Valentino et al. (2008) and Valentino and Chiarenzelli (2018). Projections of those shear zones beneath the Appalachian strata (dashed black lines) are based on Chiarenzelli and Valentino (2020). The set of blue lines in western New York is the Clarenden–Lynden fault zone, and the set of blue lines adjacent to the southern Adirondacks are faults that cut lower Ordovician, Cambrian and basement units (from Jacobi, 2002). Earthquakes are from USGS compilation span January 1920–20 January 2020 (USGS Earthquake Catalog, https://earthquake.usgs.gov/earthquakes/map/, last access: 20 November 2020; details of search listed among references cited). Induced earthquakes in western New York are attributable to brine mining-related work and to brine injection tests in an attempt to develop new caverns for gas storage (Smith et al., 2005; Horowitz et al., 2017). Polygon surrounding Ithaca delineates Tompkins County. A black line near Ithaca shows the location of a seismic reflection profile leased by Cornell to establish the distribution of faults and folds (Fig. 3).
Large areas of all continents are underlain at several kilometers' depth by mid-grade to high-grade metamorphic complexes that formed in ancient orogenic belts. Like many locations, the basement rocks anticipated in the proposed Cornell borehole will be composed of complex, heterogeneous lithologies, with anisotropic mineralogical, petrological and fabric characteristics, in which occur numerous generations of fractures that formed since peak metamorphism and are filled with multiple generations of minerals. The region is an archetypical domain of a very low level of natural seismic activity (Fig. 1) – in a circular area exceeding 13 000 km2 centered on Cornell University, there have been no earthquakes of magnitude > 2.5 in at least a century. A set of scientific experiments designed for and conducted in a deep borehole in central New York State can address questions that are generic and not specific to the eastern United States.
Integration of findings for the continental crust of New York with those expected from a similar set of experiments designed for a geothermal borehole at TU Delft in the Netherlands (Vardon et al., 2020) could, together, illuminate answers to a series of basic questions, in the interest of accelerating the safe use of subsurface thermal energy. Is the continental crust everywhere at a condition of critical stress (Zoback and Gorelick, 2012)? How heterogeneous is the stress field with depth and across lithologic boundaries? How does the subsurface, especially at the sedimentary rock–basement interface, respond to perturbations? And how will potential chemical and mineralogical reactions during operations couple to permeability, pressure transmission, and strain? Answers to these questions can provide broad insight into seismic risks and potentially how to mitigate them. Technical risks can also be addressed by determining whether the sedimentary rock–basement interface is a relevant geothermal development target and under what rock conditions artificial stimulation could improve permeability to achieve adequate fluid flow and prolonged heat transfer to permit direct use of geothermal energy where continental crust is naturally of low permeability.
The workshop was designed to maximize cross-disciplinary sharing and debate, to minimize passively listening to prepared presentations, and to lead to one or more innovative scientific projects worthy of funding. The organizers could anticipate what might be some of the big scientific themes that would become transformed into tractable borehole science projects as a result of the workshop, yet we did not wish to squelch thoughts outside of the bounds of our preconceived notions. The invitation and application process had assembled a large group with little shared background knowledge of the major questions in one another's area of interest. The focus would be a specific location with unchangeable (albeit partially unknowable) subsurface materials, and the participants would need a simple but comprehensive introduction to the materials. We did not want the group to disperse without written outcomes that would lead to submitted proposals for funds needed to conduct the science research program.
The first two days of the 3-day workshop involved 35 visitors and 26 Cornell faculty, technical staff, and students. The third day of the workshop was limited to a proposal writing team of six visitors and one of the Cornell hosts and a report writing team of two visitors and one of the Cornell hosts. The first two days were dedicated to a series of breakout sessions and plenary sessions. The single hour of background presentations conveyed a quick picture – 5 min limits – of the state of the art in themes we expected to be major science challenges and major approaches to illuminating key processes as well as the nature of the materials and structures expected in the Ithaca subsurface. A poster session was available during all breaks, and several meters of core through rocks similar to what are expected below Ithaca were on display. Even after opportunely adding three extra talks while the workshop progressed, the total structured workshop time dedicated to slide presentations was about 8 %. There were three length exceptions, yet only 15 min each: Cornell's lead engineer in the Earth Source Heat project team, the Dean of Engineering, and representatives of the U.S. Continental Scientific Drilling Program and the New York State Museum. The latter short presentation introduced the materials, services, and protocols for data and samples that are hosted by their organizations and available for integration into a proposed Cornell-based test borehole scientific program.
In the remaining > 90 % of the time, the dominant activities were breakout discussions interspersed with plenary discussions. The first breakout groups were seeded with five topics, populated by self-selected participants, and dedicated to brainstorming. The second breakout activity moved the regional geological experts into the other groups, as a knowledge resource. In the third breakout session, the geologically oriented group reconvened while the drilling group disbanded, and those experts placed their drilling and borehole management experience in the science-focused breakouts. Through that series of slightly shifting breakout themes and participants and intervening plenary discussions, the interest groups evolved from laudatory-but-impractical dreams to a more realistic borehole project design. On the second day, new breakout groups were constituted based on expertise rather than interest, and these groups critiqued and refined the research approaches suggested by the first-day groups. A session was dedicated to considering the operational coexistence that would be needed between the scientific projects and the Cornell ESH borehole team, whose focus would be on managing risk, minimizing costs, and achieving the practical goals of the borehole. In anticipation that funds needed for some of the scientific experiments would need to be raised from multiple funding sources and that principal investigators behind these complementary projects would not necessarily be Cornell University personnel, a hypothetical organizational structure to coordinate and evaluate proposed borehole sampling and experiments was unveiled and critiqued, benefitting from feedback from participants with experience in other collaborative natural laboratories.
Undoubtedly this workshop structure would have appeared to be quite messy if one were watching the events. Yet the outcome was that the full group of participants was energetically involved and learning from one another at a high rate. The theme area interest groups as well as the workshop group as a whole moved forward to create an integrated science plan and experimental framework that became physically realistic, albeit complex and challenging.
Existing borehole and geophysical data for New York State indicate that the Ithaca area basement rocks are likely similar to those which crop out in the Adirondack Mountains (Fig. 1), which is a Grenville age mid- to high-grade metamorphic dome. We expect the basement to be a mixture of metasedimentary and meta-igneous rocks, repeatedly folded while ductile (McLelland et al., 2010; Chiarenzelli et al., 2011; Valentino et al., 2019). If represented by the Adirondack Mountains, the petrological heterogeneity likely spans marbles to anorthosite, with compositionally variable gneisses and schists. Cuttings and kimberlite xenocrysts (Kay et al., 1983) in the region near Cornell University confirm this degree of variability.
The length scale of compositional heterogeneity in the basement likely spans centimeters to tens of kilometers, and there would likely be strong anisotropy of metamorphic fabrics at scales of millimeters to kilometers. Superimposed brittle fractures in the Adirondacks are abundant, with dominant orientations trending NE and NW, and most are filled with mineral veins (Wiener and Isachsen, 1987; Valentino et al., 2016). Whether any of the outcrop fracture properties are applicable to the deep subsurface of the Appalachian Basin is unknown.
The dominant sedimentary rocks near Cornell are an upward progression from basal sandstones, to carbonates, and to siliciclastic mudstone and sandstone, interrupted by hundreds of meters of Silurian evaporite deposits, dolomite, and mudstones (Salina Group) and by two intervals of organic-rich shales (Fig. 2). The best-documented structures near Ithaca are of two types. First, there are small-magnitude extensional or transtensional fault sets localized in narrow, shallow grabens (Fig. 3), known well for hosting major gas fields (Smith, 2006). The second type of structures are small magnitude folds and thrusts of the distal Alleghanian Orogeny, localized within and above the weak shales and evaporites of the Silurian section (Fig. 3).
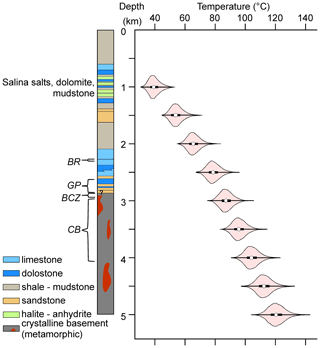
Figure 2Left: approximate geological column near Ithaca, NY. Paleozoic sedimentary rocks extend to about 2850 m depth ±200 m, underlain by metamorphic basement. Targeted intervals to probe for their geothermal reservoir conditions are indicated by brackets, corresponding to the BR (Black River), GP (Galway and Potsdam), BCZ (basement contact zone), and CB (crystalline basement). Cuttings from 46 m (150 ft) penetration into the basement by a borehole 10 km south of Cornell indicate a hydrothermally altered granitic to monzonitic gneiss (Benjamin R. Valentino, unpublished data, 2016). Near Cornell's campus, xenocrysts in kimberlites are very rich in Mg, suggestive of granulite-grade marble in the upper basement (Kay et al., 1983). Right: predicted temperature–depth profile below Cornell University in Ithaca, NY, based on geostatistical interpolation of the surface heat flow as estimated from corrected borehole temperatures in the Appalachian Basin near Ithaca, utilizing 10 000 Monte Carlo replications to incorporate the uncertainty in geologic properties and heat flow estimates (Smith, 2019). The uncertainty distribution of predicted temperatures for each 0.5 km depth interval is shown as a pale red violin plot (kernel density plot). White circles are placed at the median predicted temperature at each depth, and a narrow black box in the center spans the 25th to 75th percentile estimates.
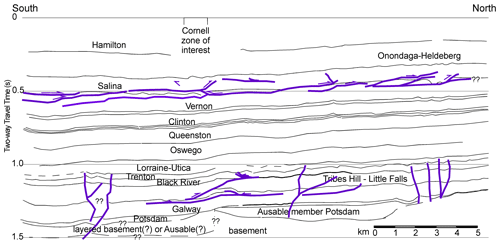
Figure 3Line drawing of an interpreted seismic reflection profile (location in Fig. 1) which traverses a short distance east of Cornell's targeted geothermal well field area of interest. Black thin lines are stratigraphic contacts within the sedimentary rock column. Purple thick lines are faults. Near 0.5 s TWTT, the set of faults are thrusts within the weak series of Silurian mudstones and evaporites. Between 1.0 and 1.5 s TWTT the faults are of two classes. The small-offset sub-vertical sets are typical of the Trenton–Black River grabens, related to wrench faults. The sub-horizontal faults are small displacement thrusts. Both the shallow and deep thrusts likely were active during the Alleghanian Orogeny. Interpretation by Jordan (2019).
Thermochronological data imply that Cornell's near-surface strata were originally covered by 3 ± 1 km thickness of Carboniferous and Permian strata, all of which were erosionally removed (Miller and Duddy, 1989; Roden and Miller, 1989; Heizler and Harrison, 1998; Shorten and Fitzgerald, 2019). During Paleozoic deposition and the subsequent long hiatus, today's preserved strata experienced extensive compaction and cementation, which rendered them strong. With few exceptions, the sedimentary rocks of the deepest 1 km of the Appalachian Basin in New York State have porosity < 10 %, and the dominant porosity is < 5 % (Martin, 2011; Al Aswad, 2019). Localized higher fracture porosity is inferred but not measured (Martin, 2011). In general, there is a lack of permeability data. We deduce that permeability for this rock interval must be less than in productive gas fields north and west of Cornell, where the best values range from 0.1 to 4 millidarcy (10−16–10−15 m2) (Lugert et al., 2006), and therefore permeability of most of the deep strata is likely on the order of microdarcy (10−18 m2). Near Cornell, the known exceptions occur in narrow, localized pods of hydrothermal dolomite associated with grabens, where much higher porosity and good permeability occur within a single 30 m thick interval about 500 m above the basement (Smith 2006; Camp and Jordan, 2016).
Available data suggest that the porewater throughout the column of sedimentary rocks possesses a summed Na and Cl concentration between 200 000 and 300 000 mg L−1 (Waller et al., 1978; Lynch and Castor, 1983; Blondes et al., 2017). Although very saline, the brine composition is within the range of basinal brines known globally (Houston et al., 2011). The pore fluids in the crystalline basement rocks are not documented; they might be similarly concentrated brines with somewhat distinctive compositions (e.g., Frape et al., 1984) or they might be much less saline.
Ithaca has at least one highly unusual geological feature, which likely will not generalize to most other continental crust: very narrow (centimeter to meter) late Mesozoic kimberlite dikes are abundant in north-trending fractures in the Ithaca region (Fig. 3) (Kay et al., 1983; Bailey et al., 2017). Limited geochronological data indicate that dike intrusion occurred around 146–148 Ma (U–Pb ages; Heaman and Kjarsgaard, 2000) or in two clusters between 113 and 146 Ma (K–Ar ages) (Basu et al., 1984; Bailey and Lupulescu, 2015). The heat pulse associated with the kimberlites decayed long ago.
The deep geology below Ithaca also has numerous uncertainties. One category of uncertainty is natural fractures at depths below the weak mudrocks and evaporites of the Silurian (Fig. 3). Another uncertainty is the nature of features that control gentle folds deep in the sedimentary column (Fig. 3). Whether these are related to inherited topography at the top of the crystalline basement or represent reactivation of basement faults during the Alleghanian Orogeny cannot be resolved with the available subsurface data. These uncertainties likely contribute to the commonality with other potential geothermal energy sites in continental interiors – there are always unknowns regarding the mechanical conditions and heterogeneities within the subsurface.
5.1 Multiple, complementary boreholes improve the scientific value of a project
A strategy for a scientific borehole mission at Cornell University must be placed in the context of coexistence with a pair of boreholes planned to demonstrate the viability of producing geothermal fluid at a sufficient flow to meet 20 % of Cornell's building heat needs. Those demonstration boreholes must be wide enough in diameter to sustain production at a flow rate on the order of 30–70 kg s−1; typically, due to this flow rate, geothermal production wells are wider in diameter than used for the oil and gas industry, groundwater production, or mineral exploration. Hence careful consideration is needed of what types of in-borehole activities and monitors are compatible with the needs of a well that eventually may function as either an injection or withdrawal well. Given that the geothermal demonstration wells would be wide in diameter, cased through intervals that present environmental or borehole management risks, maintained in a disturbed condition if production is initiated, and necessarily that their operations would prioritize the success of the geothermal project, there would be both short-term design and long-term management challenges related to co-located scientific program activities. The design and management challenges for scientific activities include the following general possibilities: interruptions to the higher-priority geothermal-focused activities; costly delays in higher-priority activities; technical incompatibility between methods of anchoring permanent monitoring equipment and casing programs; and the potential for stuck tools or borehole wall damage or collapse, which place future access and use of the borehole at risk of failure.
The scientific and geothermal demonstration boreholes are unambiguously complementary to one another in their missions, data streams, and outcomes. Nevertheless, discussions at the ICDP Workshop of efforts to complete in the same borehole both programs, science and geothermal operations, repeatedly arrived at concerns that workarounds to do both would create a higher-priced, technically more challenging borehole program with higher overall likelihood of technical failure.
An alternative is to design the initial geothermal borehole demonstration project as a three-hole system (Fig. 4). A first stage would include two boreholes. Hole 1A would be a slimmer diameter hole to the top of the basement and a short distance into the basement, preferably 100–200 m, from which an extensive rock core would be extracted. Hole 1B would follow soon thereafter and be a borehole of wide enough diameter to potentially be used for well field extraction or injection and drilled to the intended project total depth, preferably 1–2 km into the crystalline basement. Hole 1A would serve as a dedicated exploratory/observation hole in which investigations would be undertaken to address uncertainties regarding hydrologic properties and conditions, thermal resource potential, coupled thermo–hydro–chemical–mechanical behavior, and chemical reactivity. These studies, complemented by geophysical and geologic investigations and modeling analysis, would provide important insights for designing subsequent production wells and help reduce risk for the project overall.
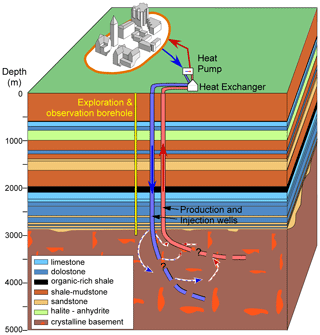
Figure 4Schematic of the geology beneath Cornell and of the three boreholes recommended by the workshop exercise. An initial borehole is recommended as stage 1A (yellow well), which would be dedicated to collection of samples and data and later be transformed into a long-term observatory. Holes 1B and 2 are the other two wells illustrated. Those pilot demonstration wells would be of wider diameter, adequate for producing or injecting the large-volume flux needed for a successful geothermal heat project. Perturbations of the near-field subsurface by drilling, testing, stimulating, and producing the wide-diameter wells would be monitored by equipment installed in the smaller diameter borehole as well as by seismometers at the surface and in shallow boreholes.
In the short term, Hole 1A would be characterized with a battery of geophysical wireline logs to directly measure physical properties, characterize fractures, orient core, and indicate stress, and a vertical seismic profile to tie core and reflection seismic depths (Table 1). Prior to completion, quantitative measures of in situ liquid transmissivity and stress at successive depths are necessary to characterize the reservoir and assist in the assessment of seismic risk. During completion, Hole 1A would be equipped with fiber optic cables to allow for short- and long-term monitoring of the vertical profile of temperature and strain, with seismometers and with pressure sensors. Hole 1A would be available for subsequent geophysical investigations intended to assist in planning for the production drilling and for longer-term monitoring of the disturbed rock mass.
By having a dedicated observation hole, borehole investigations (including and beyond those in Table 1) are possible which could provide broadly applicable scientific insights. For example, an understanding of the thermal and mineralogic history of deep geology within the region can be assessed, the deep microbiology can be sampled and evaluated, and the coupled thermo–hydro–chemical–mechanical processes associated with subsurface perturbations can be monitored, analyzed, and compared with other geologic settings around the world.
A key part of the completion of Hole 1A will be maintaining, ideally for many years, some direct access to the reservoir to allow for direct sampling of fluids and the monitoring of pressures and productivity during the later production. The preliminary results from Hole 1A would be used to finalize the drilling plan for the first production-diameter Hole 1B.
The exploratory borehole would be designed to be vertical to facilitate testing and stratigraphic mapping. In contrast, the production demonstration borehole might intentionally deviate from vertical in order to optimally access the reservoir, or it might involve laterals.
Once both Holes 1A and 1B exist, inter-borehole communication of fluids, pressure, and temperature can be investigated. After formation tests and fracture tests are performed using one or both of these boreholes, the need for a stimulation plan to develop sufficient fracture permeability to maintain viable heat production would be re-evaluated and the detailed plan finalized and conducted. Then the second stage would begin, the drilling of another production Hole 2, planned to land within the zone of fractures stimulated from the 1B borehole.
Whichever borehole plan advances, it will be accompanied by monitoring of seismic activity using a local seismic array that has been operational since 2019 and that might be densified during drilling and testing. Furthermore, group discussions revealed that there is considerable value to complementing the borehole observations with advanced seismic probing of the area near the proposed geothermal well field. Seismic fracture mapping (Sicking and Malin, 2019) offers the possibility of independent documentation of the spatial variability of permeability in the rock volume, and a three-dimensional seismic reflection profile would enhance understanding and modeling of the shape of the sedimentary rock–basement interface, with its key mechanical role. Although these advanced seismic experiments might be conducted prior to drilling the first well, alternatively they could be conducted in the months between boring Holes 1A and 1B and therefore take advantage of newly available sonic velocity data from the borehole and of the potential to combine surface seismic nodes with instruments in Hole 1A.
The workshop led to an appreciation that the scientific value of subsurface studies would be greatly enhanced by including both the small diameter Hole 1A and demonstration project Hole 1B. The core scientific problems involve the behavior of a volume of rock: the coupled responses among stress, strain, mineralogy and chemistry of the rock-fluid system in the volume when perturbed. Given a single borehole as the initial experiment, the information derived from tests would inform us of a single vertical column of rock, whose horizontal dimension is limited to a few square meters. If instead there are two initial boreholes (1A, 1B), the logging data set and active tests will document the properties, conditions, and heterogeneity of a more representative rock volume.
Even more importantly, Hole 1A would be a relatively inexpensive investment to reduce subsequent risks during drilling of the more costly production holes. First, documentation of the temperature profile below Cornell would remove the uncertainty on existing temperature estimates (Fig. 2) which have been used as the basis for assessing the economic viability of a geothermal well field. Second, conducting much of the logging and coring in the comparatively slim hole would greatly reduce the risk of damage or borehole loss to the first demonstration well. Third, data and insight from an integrated package of samples and rock property logs and tests in Hole 1A would allow refinement of the drilling and casing plan for the primary, wide borehole (Hole 1B). Fourth, while formation tests and fracture tests are conducted in the broad diameter well, simultaneous observations in the observation Hole 1A would underpin better interpretations on which further major decisions (preparations for stage 2) will rely. In combination with geophysical data collected in paired borehole–surface experiments, the heterogeneity, vertically and horizontally, of the first two wells would lay the foundation for characterizing the anisotropy of rock, fractures and fluids prior to drilling the third well. This 3-D information would improve models of what will occur during stimulation and inform the design of a third borehole. Having a firm basis in advance for those models would improve the value of the exercise of model validation through comparison of fractures and fluids in the third borehole to the models and comparison of modeled stimulation to true stimulation data.
5.2 Unique properties of the proposed borehole(s) location
Counterintuitively, the lack of anything “special” about a central New York State borehole site is one of the most compelling attributes of this project. To date, scientific boreholes in continental crust have been selected to investigate active tectonic (e.g., Wenchuan Fault, Alpine Fault, San Andreas Fault, East African rift zone; Mori and Ellsworth, 2014) or volcanic systems (e.g., Iceland's Krafla volcano, Japan's Unzen volcano, the US's Yellowstone plume; Kukkonen and Fridleifsson, 2014), or very rare but large ancient cataclysmic events like meteorite impacts (e.g., Chicxulub, Chesapeake Bay; Koeberl and Claeys, 2014). Only the hydrocarbon industry, waste disposal industry, and mining industry drill several-kilometer boreholes into “normal” crust, and those wells focus on a commercial purpose with few or no opportunities for experiments to probe basic scientific questions. This leaves the Earth sciences community with large gaps in understanding of the “average” intraplate continental crust. Boring (drilling) and investigating a “boring” location is in fact novel.
The number of boreholes drilled for scientific purposes or used as holes of opportunity in “boring” older cratons remains very small. That said, such boreholes, which include the Kola “superdeep” borehole in the former Soviet Union, the German KTB project, the Finnish Outokumpu drilling, and most recently the Swedish Scientific Drilling Program, have overturned many of the long-standing presumptions of the characteristics of the crust. Specific examples of breakthroughs include the topics of the existence of fluids (e.g., Lodemann et al., 1997; Smithson et al., 2000), fluid chemistry (e.g., Kietäväinen et al., 2013), gas compositions (e.g., Wiersberg et al., 2020) and seismic characterization (e.g., Simon et al., 2017; Schijns et al., 2012). The Swedish program completed two boreholes, COSC-1 to nearly 2500 m depth in late 2014 and COSC-2 to 2276 m in 2020. Although the scientific goals of the Swedish boreholes differ, their experiences will be invaluable in planning and deploying Hole 1A.
A 2014 summary of completed ICDP projects (de Wit, 2014) illuminated that the proposed Cornell borehole, planned to traverse Devonian through Cambrian strata and continue well into rocks that achieved their mid- to high-grade metamorphic status 1000–1350 Ma, also targets an interval of Earth history that has been ignored in all but two ICDP boreholes.
Cornell University is committed to broad and rapid public dissemination of all data extracted from the borehole. Rather than the development of tools or methods hoped to provide industrial and business advantages, as might be common in some boreholes with experimental activities, the university's intent is that the borehole activities and data be widely disseminated. The workshop served as the initial opportunity to consider some models for archiving and distributing samples and data, such as the usual procedures recommended by ICDP and IODP.
Furthermore, the willingness of Cornell to host a scientific borehole at the initiation of the large-scale geothermal energy experiment provides the opportunity for characterization and monitoring of the natural, unperturbed subsurface state over a large depth range and then to continuously measure natural and human-perturbed changes over time. If appropriately designed and installed, the proposed Cornell borehole set would measure field-scale characteristics, and its findings can be integrated with what is learned in mesoscale underground laboratories that enable and host long-term monitoring, such as the Sanford Underground Research Facility (South Dakota, USA) and Bedretto Underground Laboratory (southern Switzerland).
5.3 The natural organization of scientific themes into an integrated borehole program
The workshop participants focused largely on research themes that related to Earth's internal dynamic activities, a major emphasis for the ICDP program. Three major themes are tightly connected: fluids and elemental cycling, seismicity across a range of length scales, and controlling subsurface fractures and fluid flow. These themes would likely be at the heart of a project that is well positioned to garner sufficient funds to carry out a deep borehole program. Two other major themes, of deep life and of the thermal–chemical–mineralogical evolution from Proterozoic through Phanerozoic times, excited many participants. While it is perceived that these two themes are unlikely to independently attract sufficient funds to drill a borehole, the workshop group welcomed the beneficial knowledge of deep life and deep history that can be extracted from samples and conditions at a borehole.
5.3.1 Fluid cycling, fractures, seismicity, and working constructively with the subsurface to harness geothermal energy
In low-porosity sedimentary rocks and in mid- to high-grade metamorphic rocks, what is the natural state of fluid cycling and of fractures, and how would the extent and variability of fractures determine the fluid flow through fracture permeability if the system is intentionally perturbed? How do perturbations transmitted across long distances act to either restrain or cause induced earthquakes? How close to critical failure are various parts of the deep strata and the crystalline basement?
A Cornell scientific borehole project has the potential to address all of these fundamental questions regarding the stress response of low-porosity sedimentary rocks, metamorphic rocks, and their fracture systems to pressure perturbations near the interface of strata with the basement. To test the hypothesis that continental crust is everywhere critically stressed (Zoback and Gorelick, 2012), a borehole experiment should document stress, fracture, and hydrological conditions through a vertically extensive volume that transects varying rock types, and the experiment should monitor strain and flow responses to imposed perturbations (Table 1, categories 1–4). That information can be a foundation for better understanding natural intraplate earthquakes across a range of scales and induced seismicity. A Cornell scientific borehole project also has the potential to greatly reduce uncertainty on coupled thermal–hydraulic models, to guide reservoir stimulation, and to underpin a risk mitigation strategy. For heat extraction and reservoir stimulation, are there characteristic sets of fracture properties and variable responses of fractures in varying lithologies, which should influence a design to establish efficient distributed fluid flow through fracture permeability? For risk-management design, is the hypothesis that the risk of inducing felt earthquakes would decrease if a slightly under-pressured reservoir condition is created by pumping consistent with the rock-fluid-stress system? The workshop focused on how to achieve both scientific and pragmatic goals which share a mechanical–thermal–hydrological–chemical system.
Analyses of induced earthquakes over the last decade have illuminated major uncertainties about rock response to stress and fluid flow near the interface between sedimentary rocks and their crystalline basement. Fluid pressure changes can influence the stress state and failure of rocks through the direct influence of pore pressure and through poroelastic strain effects. Using a global data set of seismic events around single injection wells and their spatial and temporal patterns, Goebel and Brodsky (2018) deduced that these seismic events reflect both induced pore-pressure changes and poroelastic coupling of the rock framework and that the contributions of those two phenomena differ between the cases of injection into “soft” sedimentary rocks compared to crystalline basement rocks. For injection into crystalline basement rocks, the detected fracture slip fits the prediction that the slip results from stress perturbation across faults close to failure that are in direct communication with fluid migration. On the other hand, injection into sedimentary rocks generated not only seismic events due to that direct pore-pressure effect, but also a much wider and longer-lasting field of slipping fractures that reflected the poroelastic response of the stress field within both the sedimentary units and underlying basement. Furthermore, Goebel and Brodsky (2018) attribute the distinction between sedimentary and basement rock behavior to the bulk elasticity, and they relate the different elasticity to porosity, which is generally higher for sedimentary than crystalline basement rocks. It is an open question whether all sedimentary rocks are essentially equal in this behavior or whether low-permeability rocks like those of an old, deeply buried sedimentary basin would transmit stress and fluids differently than more porous strata. Goebel and Brodsky (2018) consider the permeability differences to be of second-order importance, although indeed permeability influences the spatial extent reached by injected fluid. Studies such as those conducted by the National Research Council (2013) emphasize the role of faults and fractures as avenues for fluid flow, and even mineral-filled fractures of certain compositions (e.g., clays) are credited with efficient transmission of pore fluid pressure (Gray, 2017).
The potential that the sedimentary rock–crystalline basement interface region plays a non-linear role in the coupling of stress, fluids, strain and earthquakes led the ICDP-sponsored workshop participants to prioritize extensive sampling and testing in the deeper sector of the strata and the upper hundred meters of the basement.
5.3.2 The ubiquitous hidden biosphere
The deep biosphere remains little known, though it is anticipated that it participates in the natural cycles of elements (Pedersen, 2000). Limits to life may be dictated by high temperature, nutrient scarcity, high pressure, and/or extreme salinity, all of which are probable in a Cornell-located borehole. Even if the absolute limit of life has not been crossed in the rocks near the bottom of the proposed borehole, the energy available to maintain life will likely be extraordinarily limited and of uncertain origin, with possibilities inclusive of the solid organic matter in rock, chemical reactions, seismic processes and radioactive decay (Lever et al., 2015). To date, the deepest documented microbes have been found deeper than 3 km and at temperatures up to about 100 ∘C (Magnabosco et al., 2018). Whereas the known temperature range of life spans −40 to 122 ∘C (Takai et al., 2008), microbiologists anticipate that basic constituents of life, like RNA and amino acids, fail at high temperatures (Lever et al., 2015) and hence expect that temperature will determine one of the limits of life. The proposed borehole will intercept rocks below 2 km depth and will access rock temperatures around 100 ∘C and possibly higher, with extremely saline brines in the pores of the sedimentary rocks and unknown pore fluid compositions in the basement rocks. These gradients and properties create the opportunity to investigate the nature of microbes across a temperature gradient for which microbial activities and characteristics are hypothesized to decline and change (Table 1, category 6). If there is a pore fluid change near the sedimentary rock to basement contact, there may be major consequences for the microbial population.
Furthermore, studies to date indicate that deep microbial life could prove to be sensitive to the mineral substrate in the compositionally variable subsurface of the proposed borehole region. A review of the literature reveals functional roles for Fe- and S-bearing minerals and some clays yet also suggests that other clays and the abundant mineral-forming element aluminum inhibit some microbial species (Röling et al., 2015). The petrologically diverse sedimentary rocks to be traversed by the proposed borehole could enable examination of the microbial populations as functions of mineralogy, including strong contrasts of the limestone and dolomite intervals to the host minerals in other borehole sites whose microbiology has been examined, such as oceanic basalts (Salas et al., 2015) and granite (Swanner and Templeton, 2011). A central New York borehole also has a good likelihood of intersecting marble in the basement. Those marbles, whose pressure and temperature history and perhaps pore fluids differ markedly from the diagenetic conditions of the carbonates in the deep sedimentary units, may provide a useful opportunity to investigate contrasts between the microbial communities of sedimentary and metamorphic carbonate rocks. Despite the lack of participation in the workshop by any experts in deep microbial life, samples from this borehole could be the foundation for research to develop and test widely applicable hypotheses.
5.3.3 Tectonics and thermal history near a boundary between major Precambrian tectonic domains
Ithaca, New York, lies near the projected position of a Proterozoic tectonic domain boundary and in the heart of a region of prolific Mesozoic kimberlite dikes. Either attribute alone is a worthy point of departure for hypotheses concerning how today's heat flow evolved from the starting point of Proterozoic or Juro-Cretaceous tectonic activity.
A Mesoproterozoic crustal-scale shear zone separates much different metamorphic domains and passes from exposed (in the Adirondack Mountains) to buried (beneath the Appalachian Basin) and has been proposed to pass a short distance west of Ithaca (Fig. 1) or under Ithaca. In the Adirondacks, the boundary is wider than 20 km, characterized by high finite strain in ductile shear under granulite conditions (Valentino et al., 2019). The shear zone separates rocks of distinctive lithologies and ages, with the core of the Grenville orogenic belt to the north and west, and granitic composition rocks in the shear zone are geochemically of a magmatic arc origin. Valentino et al. (2019) interpret the shear zone to have formed by the collision of plates around the margins of the Laurentian continental block. If the shear zone extrapolation through the region near Ithaca were correct, then granulite metamorphic-grade meta-igneous rocks (anorthosite–mangerite–charnockite–granite) with scraps of dismembered metasedimentary rocks may be likely northwest or west of Ithaca, whereas granulite-grade tonalitic gneisses and complex metasedimentary rocks may be expected in Ithaca as well as in the south and east. Borehole samples would enable investigation of compositions, geochemistry, and geochronology with which to test the hypothesis that a terrane boundary separates Ithaca from the main Grenville orogenic core.
Kimberlite dikes are so abundant (Kay et al., 1983; Bailey et al., 2017) in north-trending fractures in the Ithaca region that there is a high probability of encountering one or more dikes in a 4 km long borehole, especially if it is deviated from vertical. If so, xenoliths and xenocrysts will provide evidence of the composition of the crustal rock through which the kimberlite arose, and populations of kimberlite xenoliths and xenocrysts collected deep in a borehole will be less diluted by the column of sedimentary rocks than are kimberlite samples studied to date. Crustal materials in the kimberlites may enable a better interpretation of the local gravity and magnetic anomalies and in turn may provide insight into the spatial heterogeneity of crustal composition. Many questions remain about the cause of the abundant kimberlites. Geochemical and thermobarometric studies of kimberlite samples should provide evidence about the magma source(s), some 150 km deep in the mantle (Kay et al., 1983). These early Cretaceous high-velocity intrusions reflect a history of paleo-strain. Can the variability of locations of kimberlites and their xenolith compositions illuminate the variability of strain responses among the various types of basement lithologies?
Shorten and Fitzgerald (2019) demonstrated that a transition occurred approximately 145 Ma from a long interval of rapid exhumation and cooling to conditions of slow exhumation and cooling. This inflection is approximately coeval with kimberlite intrusions in the earliest Cretaceous. An integrated thermochronological study of a vertical profile of borehole materials (Table 1, category 5), including kimberlites at one or more depth, offers the possibility of exploring the modern retention of low-temperature features across the modern-geothermal gradient and the opportunity to examine the post-emplacement thermal history of kimberlites where they have not been exposed to near-surface weathering.
5.3.4 Summary of scientific themes
All of these research themes are interconnected. For example, fractures and fluid migration are related to the mechanical and lithological heterogeneity that was instituted by both Precambrian orogenic activity and Phanerozoic structural adjustments to near-field and far-field phenomena. Integrated borehole measurements and experiments will reveal how the preexisting mechanical and lithological heterogeneity controls and interacts with stress and about the nature of active processes that link thermal, hydrological, and chemical processes to mechanics and to one another. Even the amount and nature of life in the deep strata and crystalline basement are likely to be linked to Precambrian–Paleozoic conditions which produced the substrate in which today's life exists and to the thermo–hydro–chemical–mechanical system which is their modern environment.
The ICDP-supported borehole planning workshop held during January 2020 in Ithaca, New York, USA, was an efficient and effective forum through which to advance the strategy for and design of a scientific borehole in continental crust. The workshop participants transformed a skeletal idea – an opportunity to utilize a proposed geothermal demonstration borehole for scientific research – into a much better developed scientific vision and the beginning of a pragmatic scientific and engineering plan. The diverse experiences and deep expertise of the workshop participants included specializations ranging from regional geology to experimental rock mechanics to geothermal borehole engineering to fracture stimulation to hydrogeology to earthquake seismology, and beyond. Collectively, this group honed a plan suitable to the northern Appalachian Basin which will illuminate how the pre-existing mechanical and lithological heterogeneity controls and interacts with stress. The discussions led to a conviction that the planned geothermal demonstration project can better encourage wide deployment of low-temperature geothermal energy technology, one of Cornell's goals, by utilizing scientific borehole studies to deepen understanding of rock behavior in the deep subsurface before and during well-field operations.
The borehole science plan that evolved (Table 1) will be costly. Ultimately, as the Cornell University drilling plan unfolds in the next few years, its features will be constrained by the details of costs, by the funds available from sources such as scientific consortia, government agencies and donors, and by initial results. A proposal to the U.S. Department of Energy that was submitted after the workshop was selected to be funded, hence Hole 1A is now in the planning phase with a drilling target of 2021. Beyond Cornell's research and geothermal demonstration plans, there will be lasting value if the essence of the integrated plan to advance understanding of thermal–hydrological–mechanical–chemical behavior of old continental crust is used by other groups at different locations.
No data sets were used in this article.
Teresa Jordan (Cornell University, USA), Patrick Fulton (Cornell University, USA), Jefferson Tester (Cornell University, USA), David Bruhn (Technical University Delft, the Netherlands/GFZ, Germany), Hiroshi Asanuma (National Institute of Advanced Industrial Sciences and Technology, Japan), Noriyoshi Tsuchiya (Tohoku University, Japan), Shigemi Naganawa (Akita University, Japan), Frederic Guinot (From Bottom to Top SàRL, Geneva, Switzerland), Hannes Hofman (GFZ Potsdam, Germany), Thomas Neumann (Technical University Berlin, Germany), Phil Vardon (TU Delft, the Netherlands), Ulrich Harms (ICDP/GFZ, Potsdam, German), Xiaodong Ma (ETH Zurich, Switzerland), Kazuya Ishitsuka (Kyoto University, Japan), Fuqiong Huang (China Earthquake Networks Center, China), Anders Noren (Continental Scientific Drilling Coordination Office, University of Minnesota, USA), Tom Pasquini (Kingwood, Texas, USA), Dorivaldo Santos (Pennsylvania State University, USA), Jared Smith (University of Virginia, USA), Trenton Cladouhos (Cyrq Energy Inc., USA), Joseph Moore (University of Utah, USA), Andy Barbour (U.S. Geological Survey, USA), Thomas Goebel (University of Memphis, USA), Nicholas Davatzes (Temple University, USA), Stephen E. Laubach (University of Texas at Austin, USA), Douglas Schmitt (Purdue University, USA), Chaoyi Wang (Purdue University, USA), David W. Valentino (State University of New York at Oswego, USA), Jeffrey R. Chiarenzelli (St. Lawrence University, USA), Brian Slater (New York State Museum, USA), Derek Elsworth (Pennsylvania State University, USA), Srisharan Shreedharan (Pennsylvania State University, USA), Suzanne Baldwin (Syracuse University, USA), Paul Fitzgerald (Syracuse University, USA), David M. Jenkins (Binghamton University USA), Natalia Zakharova (Central Michigan University, USA), Tieyuan Zhu (Pennsylvania State University, USA), Robert R. Bland (Cornell University, USA), Rick Burgess (Cornell University, USA), Larry Brown (Cornell University, USA), Matthew Pritchard (Cornell University, USA), Richard Allmendinger (Cornell University, USA), J. Olaf Gustafson (Cornell University, USA), Steve Beyers (Cornell University, USA), Sriramya Nair (Cornell University, USA), Greg McLaskey (Cornell University, USA), Greeshma Gadikota (Cornell University, USA), Suzanne M. Kay (Cornell University, USA), Frank Horowitz (Cornell University, USA), Anthony R. Ingraffea (Cornell University, USA), Nicolas Rangel Jurado (Cornell University, USA), Muawia Barazangi (Cornell University, USA), Jacob Simon (Cornell University, USA), Ivanakbar Purwamaska (Cornell University, USA), Jacob Paul (Cornell University, USA), Karin Olson Hoal (Cornell University, USA), David Hysell (Cornell University, USA), Lynden A. Archer (Cornell University, USA), and Larry Cathles (Cornell University, USA).
TJ, UH and CW framed the paper and wrote initial text at the conclusion of the workshop. TJ, PF and JT completed the initial draft. DB, HA, DS, PJV, HH, TP, and JS made substantial suggestions and additions that led to the finalized manuscript. TJ prepared the illustrations.
All but one author declares they have no conflict of interest. Ulrich Harms is the Executive Secretary and Head of Operational Support Group for the ICDP, one of the parent organizations of Scientific Drilling.
The workshop organizers and participants greatly appreciated the ICDP's support and guidance. We appreciate greatly the support of the Cornell University Departments of Chemical and Biomolecular Engineering and of Earth and Atmospheric Sciences in the form of extensive organizational efforts by staff professionals Polly Marion and Carolyn Headlam and the participation of Lance Collins, Dean of Engineering. We thank the New York State Museum, Cyrq Energy, Inc., U.S. Geological Survey and U.S. Department of Energy, which each supported the participation by an individual, and New York State Museum for the loan of core. Koenraad Beckers is thanked for correspondence and data regarding Europe and Russia's energy utilization. John Shervais and John Eichelberger are thanked for their comments on an earlier draft of this paper.
This research has been supported by the International Continental Scientific Drilling Program (grant no. 11-2019).
This paper was edited by Tomoaki Morishita and reviewed by John Eichelberger and John Shervais.
Al Aswad, J. A.: A Stratigraphic and Petrophysical Study of In-situ Geothermal Reservoir Quality of the Cambro-Ordovician Strata in the Subsurface at Cornell University, Ithaca, New York, MS Thesis, Ithaca, New York, USA, 1–172, 2019.
Bailey, D. G. and Lupulescu, M. V.: Spatial, temporal, mineralogical, and compositional variations in Mesozoic kimberlitic magmatism in New York State, Lithos, 212, 298–310, 2015.
Bailey, D. G., Lupulescu, M. V., and Chiarenzelli, J. R.: Kimberlites in the Cayuga Lake region of central New York: The Six Mile Creek, Williams Brook, and Taughannock Creek dikes, in: New York Geological Association 89th Annual Meeting Field Trip Guidebook, edited by: Muller, O. H., Alfred University, Alfred, New York, USA, 160–190, 2017.
Basu, A. R., Rubury, E., Mehnert, H., and Tatsumoto, M.: Sm-Nd, K-Ar and petrologic study of some kimberlites from eastern United States and their implication for mantle evolution, Contrib. Mineral. Petr., 86, 35–44, 1984.
Blondes, M. S., Gans, K. D., Engle, M. A., Kharaka, Y. K., Reidy, M. E., Saraswathula, V., Thordsen, J. J., Rowan, E. L., and Morrissey, E. A.: US Geological Survey National Produced Waters Geochemical Database v2. 3 (PROVISIONAL), US Geological Survey: US Geological Survey, Eastern Energy Resources Science Center, Reston, VA, USA, available at: https://eerscmap.usgs.gov/pwapp/ (last access: 23 October 2019), 2017.
Camp, E. and Jordan, T.: Feasibility study of repurposing Trenton–Black River gas fields for geothermal heat extraction, southern New York, Geosphere, 13, GES01230-1-14, https://doi.org/10.1130/GES01230.1, 2016.
Camp, E. R., Jordan, T. E., Hornbach, M. J., and Whealton, C. A.: A probabilistic application of oil and gas data for exploration stage geothermal reservoir assessment in the Appalachian Basin, Geothermics, 71, 187–199, https://doi.org/10.1016/j.geothermics.2017.09.001, 2018.
Chiarenzelli, J. R. and Valentino, D. W.: Exposed basement rock and geophysical trends in New York State: poster presentation at ICDP Workshop at Cornell University, 8–9 January 2020, Ithaca, New York, USA, 2020.
Chiarenzelli, J., Valentino, D., Lupulescu, M., Thern, E., and Johnston, S.: Differentiating Shawinigan and Ottawan orogenesis in the central Adirondacks, Geosphere, 7, 2–22, 2011.
de Wit, M.: The science plan – preamble, in: Unravelling the Workings of Planet Earth, Science Plan for 2014–2019, edited by: Horsfield, B., Knebel, C., Ludden, J., and Hyndman, R., International Continental Scientific Drilling Program, Potsdam, Germany, 18–22, 2014.
Dillinger, A., Ricard, L. P., Huddlestone-Holmes, C., and Esteban, L.: Impact of diagenesis on reservoir quality in a sedimentary geothermal play: a case study in the Cooper Basin, South Australia, Basin Res., 26, 252–272, 2016.
EIA: State energy data systems (SEDS): 1960–2016 (complete), U.S. Energy Information Administration, available at: https://www.eia.gov/state/seds/ (last access: 20 November 2020), 2018a.
EIA: 2015 Residential energy consumption survey (RECS), U.S. Energy Information Administration, available at: https://www.eia.gov/consumption/residential/data/2015 (last access: 20 November 2020), 2018b.
EIA: 2012, Commercial buildings energy consumption survey (CBECS), U.S. Energy Information Administration, available at: https://www.eia.gov/consumption/commercial/data/2012 (last access: 20 November 2020), 2019.
Frape, S. K., Fritz, P., and McNutt, R. H. T.: Water-rock interaction and chemistry of groundwaters from the Canadian Shield, Geochim. Cosmochim. Ac., 48, 1617–1627, 1984.
Goebel, T. H. W. and Brodsky, E. E.: The spatial footprint of injection wells in a global compilation of induced earthquake sequences, Science, 361, 899–904, 2018.
Gray, I.: Effective stress in rock, Proceedings of the Eighth International Conference on Deep and High Stress Mining, 28–30 March 2017, Perth, Australia, edited by: Wesseloo, J., 199–207, 2017.
Heaman, L. M. and Kjarsgaard, B. A.: Timing of eastern North American kimberlite magmatism: continental extension of the Great Meteor hotspot track, Earth Planet. Sci. Lett., 178, 253–268, 2000.
Heizler, M. T. and Harrison, T. M.: The thermal history of the New York basement determined from 40Ar∕39Ar K-feldspar studies, J. Geophys. Res.-Sol. Ea., 103, 29795–29814, 1998.
Horowitz, F. G., Ebinger, C., and Jordan, T. E.: Identifying faults Associated with the 2001 Avoca induced (?) seismicity sequence of western New York State using potential field wavelets, AGU Fall Meeting Abstracts, 11–15 December 2017, New Orleans, Louisiana, USA, S23C-0835, 2017.
Houston, S., Smalley, C., Laycock, A., and Yardley, B. W. D.: The relative importance of buffering and brine inputs in controlling the abundance of Na and Ca in sedimentary formation waters, Mar. Petrol. Geol., 28, 1242–1251, 2011.
Jacobi, R. D.: Basement faults and seismicity in the Appalachian Basin of New York State, Tectonophysics, 353, 75–113, 2002.
Jordan, T.: Geological evaluation of subsurface features near Ithaca, NY: interpretations of seismic reflection profiles collected by the petroleum industry, eCommons, 1–44, available at: https://ecommons.cornell.edu/handle/1813/69546 (last access: 20 November 2020), 2019.
Kay, S. M., Snedden, W. T., Foster, B. P., and Kay, R. W.: Upper mantle and crustal fragments in the Ithaca kimberlites, J. Geol., 91, 277–290, 1983.
Kietäväinen, R., Ahonen, L., Kukkonen, I. T., Hendriksson, N., Nyyssönen, M., and Itävaara, M.: Characterisation and isotopic evolution of saline waters of the Outokumpu Deep Drill Hole, Finland–Implications for water origin and deep terrestrial biosphere, Appl. Geochem., 32, 37–51, 2013.
Koeberl, C. and Claeys, P.: Cataclysmic events – Impact craters and processes, in: Unravelling the Workings of Planet Earth, Science Plan for 2014–2019, edited by: Horsfield, B., Knebel, C., Ludden, J., and Hyndman, R., International Continental Scientific Drilling Program, Potsdam, Germany, 66–73, 2014.
Kukkonen, I. T. and Fridleifsson, G. O.: Heat and Mass Transfer, in: Unravelling the Workings of Planet Earth, Science Plan for 2014–2019, edited by: Horsfield, B., Knebel, C., Ludden, J., and Hyndman, R., International Continental Scientific Drilling Program, Potsdam, Germany, 42–55, 2014.
Kummu, M. and Varis, O.: The world by latitudes: A global analysis of human population, development level and environment across the north–south axis over the past half century, Appl. Geogr., 31, 495–507, 2011.
Le Brun, M., Hamm, V., Lopez, S., Ungemach, P., Antics, M., Aussear, J. Y., Cordier, E. Giuglaris, E., Goblet, P., and Lalos, P.: Hydraulic and thermal impact modeling at the scale of the geothermal heating doublet in the Paris Basin, France, Geothermal Reservoir Engineering, Thirty-Sixth Workshop, 31 January–2 February 2011, Stanford, CA, USA, SGP-TR-191, 1–14, 2011.
Lever, M. A., Rogers, K. L., Lloyd, K. G., Overmann, J., Schink, B., Thauer, R. K., Hoehler, T. M., and Jørgensen, B. B.: Life under extreme energy limitation: a synthesis of laboratory-and field-based investigations, FEMS Microbiol. Rev., 39, 688–728, 2015.
Limberger, J., Boxem, T., Pluymaekers, M., Bruhn, D., Manzella, A., Calcagno, P., Beekman, F., Cloetingh, S., and van Wees, J.-D.: Geothermal energy in deep aquifers: A global assessment of the resource base for direct heat utilization, Renew. Sust. Energ. Rev., 82, 961–975, 2018.
Lodemann, M., Fritz, P., Wolf, M., Ivanovich, M., Hansen, B. T., and Nolte, E.: On the origin of saline fluids in the KTB (continental deep drilling project of Germany), Appl. Geochem., 12, 831–849, 1997.
Lugert, C., Smith, L., Nyahay, R., Bauer, S., and Ehgartner, B.: Systematic technical innovations initiative for brine disposal in the northeast, New York State Energy Research and Development Authority Contract Number 6940, Albany, NY, USA, 1–269, 2006.
Lynch, R. S. and Castor, T. P.: Auburn Low-Temperature Geothermal Well, Final Report: NYSERDA Report 84-18, Albany, NY, USA, 1–277, 1983.
Magnabosco, C., Lin, L.-H., Dong, H., Bomberg, M., Ghiorse, W., Stan-Lotter, H., Pedersen, K., Kieft, T. L., van Heerden, E., and Onstott, T. C.: The biomass and biodiversity of the continental subsurface, Nat. Geosci., 11, 707–717, 2018.
Martin, J.: Carbon sequestration feasibility study in the Chautauqua County, New York area, New York State Energy Research and Development Authority 10498, Albany, NY, USA, 1–424, 2011.
McCabe, K., Gleason, M., Reber, T., and Young, K. R.: Characterizing U.S. heat demand for potential application of geothermal direct use, Proceedings 40th GRC Annual Meeting, 23–26 October 2016, Sacramento, CA, USA, 1–22, available at: https://www.nrel.gov/docs/fy17osti/66460.pdf (last access: 20 November 2020), 2016.
McLelland, J. M., Selleck, B. W., and Bickford, M. E.: Review of the Proterozoic evolution of the Grenville Province, its Adirondack outlier, and the Mesoproterozoic inliers of the Appalachians, in: From Rodinia to Pangea: The Lithotectonic Record of the Appalachian Region, edited by: Karabinos, P. M., Hibbard, J. P., Bartholomew, M. J., and Tollo, R. P., Geological Society of America Memoir 206, Boulder, Colorado USA, 1–29, 2010.
Miller, D. S. and Duddy, I. R.: Early Cretaceous uplift and erosion of the northern Appalachian Basin, New York, based on apatite fission track analysis, Earth Planet. Sc. Lett., 93, 35–49, 1989.
Mori, J. and Ellsworth, W.: Active Faults and Earthquakes, in: Unravelling the Workings of Planet Earth, Science Plan for 2014–2019, edited by: Horsfield, B., Knebel, C., Ludden, J., and Hyndman, R., International Continental Scientific Drilling Program, Potsdam, Germany, 24–31, 2014.
National Research Council: Induced seismicity potential in energy technologies, U.S. National Academies Press, Washington, DC, USA, https://doi.org/10.17226/13355, 2013.
NYSERDA: New York State Greenhouse Gas Inventory: 1990–2016, Final Report, New York State Energy Research and Development Authority, Albany, NY, USA, available at: https://www.nyserda.ny.gov/About/Publications/EA-Reports-and-Studies/Energy-Statistics (last access: 20 November 2020), 2019.
Pedersen, K.: Exploration of deep intraterrestrial microbial life: current perspectives, FEMS Microbiol. Lett., 185, 9–16, 2000.
Roden, M. K. and Miller, D. S.: Apatite fission-track thermochronology of the Pennsylvania Appalachian Basin, Geomorphology, 2, 39–51, 1989.
Röling, W. F. M., Aerts, J. W., Patty, C. H. L., Ten Kate, I. L., Ehrenfreund, P., and Direito, S. O. L.: The significance of microbe-mineral-biomarker interactions in the detection of life on Mars and beyond, Astrobiology, 15, 492–507, 2015.
Salas, E. C., Bhartia, R., Anderson, L., Hug, W. F., Reid, R. D., Iturrino, G., and Edwards, K. J.: In situ detection of microbial life in the deep biosphere in igneous ocean crust, Front. Microbiol., 6, 1260, https://doi.org/10.3389/fmicb.2015.01260, 2015.
Schijns, H., Schmitt, D. R., Keikkinen, P. J., and Kukkonen, I. T.: Seismic anisotropy in the crystalline upper crust: observations and modelling from the Outokumpu scientific borehole, Finland, Geophys. J. Int., 189, 541–553, 2012.
Shorten, C. M. and Fitzgerald, P. G.: Post-orogenic thermal history and exhumation of the northern Appalachian Basin: Low-temperature thermochronologic constraints, Basin Res., 31, 1017–1039, 2019.
Sicking, C. and Malin, P.: Fracture Seismic: mapping subsurface connectivity, Geosciences, 9, 1–35, 2019.
Simon, H., Buske, S., Krauss, F., Giese, R., Hedin, P., and Juhlin, C.: The derivation of an anisotropic velocity model from a combined surface and borehole seismic survey in crystalline environment at the COSC-1 borehole, central Sweden, Geophys. J. Int., 210, 1332–1346, 2017.
Smith, J. D.: Exploratory spatial data analysis and uncertainty propagation for geothermal resource assessment and reservoir models, PhD Thesis, Cornell University, Ithaca, NY, USA, 1–255, 2019.
Smith, L. B.: Origin and reservoir characteristics of Upper Ordovician Trenton-Black River hydrothermal dolomite reservoirs in New York, AAPG Bull., 90, 1691–1718, https://doi.org/10.1306/04260605078, 2006.
Smith, L., Lugert, C., Bauer, S., Ehgartner, B., and Nyahay, R.: Final Report: Systematic Technical Innovations Initiative Brine Disposal in the Northeast, National Energy Technology Lab (DOE) DE-FC26-01NT41298, Albany, New York, USA, 2005.
Smithson, S. B., Wenzel, F., Ganchin, Y. V., and Morozov, I. B.: Seismic results at Kola and KTB deep scientific boreholes: velocities, reflections, fluids, and crustal composition, Tectonophysics, 329, 301–317, 2000.
Swanner, E. and Templeton, A.: Potential for nitrogen fixation and nitrification in the granite-hosted subsurface at Henderson Mine, CO, Front. Microbiol., 2, 254, https://doi.org/10.3389/fmicb.2011.00254, 2011.
Takai, K., Nakamura, K., Toki, T., Tsunogai, U., Miyazaki, M., Miyazaki, J., Hirayama, H., Nakagawa, S., Nunoura, T., and Horikoshi, K.: Cell proliferation at 122C and isotopically heavy CH4 production by a hyperthermophilic methanogen under high-pressure cultivation, P. Natl. Acad. Sci. USA, 105, 10949–10954, 2008.
USGS Earthquake Catalog: https://tinyurl.com/yyewvgax, last access: 20 November 2020.
Valentino, D. W. and Chiarenzelli, J. R.: The Piseco Lake Shear Zone: a Shawinigan cryptic suture in the southern Adirondack Mountains, New York, New York State Geological Association, Castleton, Vermont, USA, 90, 139–156, 2018.
Valentino, D. W., Chiarenzelli, J. R., Piaschyk, D., Williams, L., and Peterson, R.: The southern Adirondack sinistral transpressive shear system, Friends of the Grenville Field Trip, Indian Lake, New York, USA, 1–56, 2008.
Valentino, D. W., Valentino, J. D., Chiarenzelli, J. R., and Inclima, R. W.: Faults and Fracture Systems in the Basement Rocks of the Adirondack Mountains, New York, Adirondack Journal of Environmental Studies, 20, 101–117, 2016.
Valentino, D. W., Chiarenzelli, J. R., and Regan, S. P.: Spatial and temporal links between Shawinigan accretionary orogenesis and massif anorthosite intrusion, southern Grenville province, New York, USA, J. Geodynam., 129, 80–97, 2019.
Vardon, P., Bruhn, D., Steiginga, A., Cox, B., Abels, H., Barnhoorn, A., Drijkoningen, G., Slob, E., and Wapenaar, K.: A Geothermal Well Doublet for Research and Heat Supply of the TU Delft Campus, arXiv [preprint], arXiv:2003.11826, 26 March 2020.
Waller, R. M., Turk, J. T., and Dingman, R. J.: Potential effects of deep-well waste disposal in Western New York, US Geol. Surv. Professional Paper 1053, Washington, DC, USA, 1–39, 1978.
Wiener, R. W. and Isachsen, Y. W.: Detailed studies of selected, well-exposed fracture zones in the Adirondack Mountains dome, New York, New York State Geological Survey, Albany, NY, USA, 1–92, 1987.
Wiersberg, T., Pierdominici, S., Lorenz, H., Almqvist, B., Klonowska, I., and C.O.S.C.S. Team: Identification of gas inflow zones in the COSC-1 borehole (Jamtland, central Sweden) by drilling mud gas monitoring, downhole geophysical logging and drill core analysis, Appl. Geochem., 114, 104513, https://doi.org/10.1016/j.apgeochem.2019.104513, 2020.
Wright, P. M. and Culver, G.: Nature of Geothermal Resources, in: Geothermal Direct Use Engineering and Design Guidebook, edited by: Lienau, P. J. and Lunis, B. C., U. S. Department of Energy Idaho Operations Office, Idaho Falls, Idaho, USA, 23–50, available at: https://inis.iaea.org/collection/NCLCollectionStore/_Public/23/044/23044859.pdf (last access: 20 November 2020), 1991.
Zoback, M. D. and Gorelick, S. M.: Earthquake triggering and large-scale geologic storage of carbon dioxide, P. Natl. Acad. Sci. USA, 109, 10164–10168, 2012.
- Abstract
- Introduction: a convergence of society's need for low-carbon heat and Earth scientists' need to understand continental crust
- Why central New York State and why now?
- Workshop structure
- Geological features of the Cornell borehole project site
- Findings
- Conclusions
- Data availability
- Team list
- Author contributions
- Competing interests
- Acknowledgements
- Financial support
- Review statement
- References
- Abstract
- Introduction: a convergence of society's need for low-carbon heat and Earth scientists' need to understand continental crust
- Why central New York State and why now?
- Workshop structure
- Geological features of the Cornell borehole project site
- Findings
- Conclusions
- Data availability
- Team list
- Author contributions
- Competing interests
- Acknowledgements
- Financial support
- Review statement
- References