the Creative Commons Attribution 4.0 License.
the Creative Commons Attribution 4.0 License.
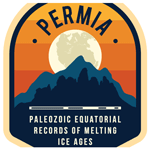
Paleozoic Equatorial Records of Melting Ice Ages (PERMIA): calibrating the pace of paleotropical environmental and ecological change during Earth's previous icehouse
Joshua M. Feinberg
Adam K. Huttenlocker
Randall B. Irmis
Declan Ramirez
Rashida Doctor
John McDaris
Charles M. Henderson
Michael T. Read
Kristina Brady Shannon
Anders Noren
Ryan O'Grady
Ayva Sloo
Patrick Steury
Diego P. Fernandez
Amy C. Henrici
Neil J. Tabor
The upper Paleozoic Cutler Group of southern Utah, USA, is a key sedimentary archive for understanding the Earth-life effects of the planet's last pre-Quaternary icehouse–hothouse state change: the Carboniferous–Permian (C–P) transition, between 304 and 290 million years ago. Within the near-paleoequatorial Cutler Group, this transition corresponds to a large-scale aridification trend, loss of aquatic habitats, and ecological shifts toward more terrestrial biota as recorded by its fossil assemblages. However, fundamental questions persist. (1) Did continental drift or shorter-term changes in glacio-eustasy, potentially driven by orbital (Milankovitch) cycles, influence environmental change at near-equatorial latitudes during the C–P climatic transition? (2) What influence did the C–P climatic transition have on the evolution of terrestrial ecosystems and on the diversity and trophic structures of terrestrial vertebrate communities?
The Paleozoic Equatorial Records of Melting Ice Ages (PERMIA) project seeks to resolve these issues in part by studying the Elk Ridge no. 1 (ER-1) core, complemented by outcrop studies. This legacy core, collected in 1981 within what is now Bears Ears National Monument, recovered a significant portion of the Hermosa Group and the overlying lower Cutler Group, making it an ideal archive for studying paleoenvironmental change during the C–P transition. As part of this project, the uppermost ∼ 450 m of the core were temporarily transferred from the Austin Core Repository Center to the Continental Scientific Drilling Facility at the University of Minnesota for splitting, imaging, and scanning for geophysical properties and spectrophotometry. Here we (1) review the history of this legacy core, (2) introduce recently obtained geophysical and lithologic datasets based on newly split and imaged core segments to provide a sedimentological and stratigraphic overview of the Elk Ridge no. 1 core that aligns more accurately with the currently recognized regional lithostratigraphic framework, (3) establish the position of the boundary between the lower Cutler beds and the overlying Cedar Mesa Sandstone in the core, and (4) outline our ongoing research goals for the core.
In-progress work on the core aims to refine biostratigraphic and chemostratigraphic age constraints, retrieve the polarity stratigraphy, interrogate preserved cyclostratigraphy, analyze sedimentary structures and paleosol facies, investigate stable isotope geochemistry, and evaluate elemental abundance data from X-ray fluorescence (XRF) scanning. Together with outcrop studies throughout Bears Ears National Monument and its vicinity, these cores will allow the rich paleontological and paleoenvironmental archives recorded in the continental Carboniferous–Permian transition of western North America to be confidently placed in a robust chronologic context that will help test hypotheses relating ecosystem evolution to the Carboniferous rainforest collapse, initial decline of the Late Paleozoic Ice Age, and long-wavelength astronomical cycles pacing global environmental change.
- Article
(7562 KB) - Full-text XML
- BibTeX
- EndNote
As global climate change impacts our present day, understanding the intricate feedback mechanisms that regulate Earth's climate has become a focal point across geoscience disciplines. In the geological past, the Late Paleozoic Ice Age (LPIA) has a special relevance to current climate studies because it serves as our only deep-time analog for comprehending “climate change in an icehouse on a vegetated Earth” (Gastaldo et al., 1996; Montañez and Soreghan, 2006). This unique episode coincided with the highest rates of organic carbon burial in the past half billion years, resulting in low atmospheric pCO2 and high pO2 levels. These conditions likely sustained the longest-lived icehouse of the Phanerozoic Eon and may have catalyzed significant evolutionary innovations in terrestrial life, including such traits as insect flight and gigantism (Berner et al., 2007; Montañez, 2016).
Towards the end of the LPIA, during the C–P transition (∼ 304–290 Ma), a climatic shift to substantially more arid conditions resulted in the dramatic recession of the formerly vast paleotropical wetlands of low-latitude western and central Pangea (Sahney et al., 2010; Pardo et al., 2019). This loss of freshwater and classic “coal swamp” habitats, conceptualized as the Carboniferous rainforest collapse hypothesis, marked a profound shift in continental ecosystems. Indeed, many paleontological studies of Late Paleozoic central Pangea repeatedly demonstrate a stark contrast between Late Carboniferous paleoequatorial ecosystems, characterized by a blend of aquatic and terrestrial species and Cisuralian (Early Permian) environments dominated by more terrestrial faunal and floral assemblages (Figs. 1–2) (Vaughn, 1966, 1970; Olson and Vaughn, 1970; Frederiksen, 1972; Rowley et al., 1985; Gastaldo et al., 1996; Cleal and Thomas, 1999; Cleal and Thomas, 2005; Pardo et al., 2019). These changes also coincide with the emergence of the first large-bodied tetrapod herbivores, a pervasive feature of terrestrial ecosystems from the Permian through to the present day (Sues and Reisz, 1998; Reisz and Sues, 2000; Pearson et al., 2013; Reisz and Fröbisch, 2014; Brocklehurst et al., 2015).
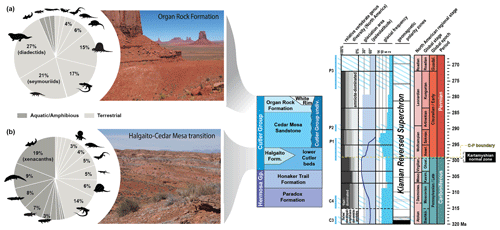
Figure 1Climate-associated shifts in paleoequatorial vertebrate diversity within the Cutler Group during the C–P transition. Vertebrate abundance in C–P Halgaito Formation (b) is biased toward aquatic taxa, whereas the Permian Organ Rock assemblage (a) is less even (differential relative abundance of each clade) and dominated by terrestrial stem-amniote herbivores (diadectids) and amniotes. This compositional change broadly coincided with the final phases of Gondwanan glaciation and desertification of western Pangea, but the ages and positions of stage boundaries in these units are poorly constrained (glacial data from Fielding et al., 2008, and Montañez and Poulsen, 2013).
Although at first glance this may suggest a mass extinction event, quantitative analysis of late Paleozoic equatorial fossil assemblages, as detailed by Pardo et al. (2019), supports the notion that this environmental and ecological shift was protracted and spatially diachronous, corroborating earlier hypotheses (Vaughn, 1966, 1970; Olson and Vaughn, 1970). This in turn led to the development of the Vaughn–Olson model (Pardo et al., 2019), which posits that the proliferation of terrestrial faunas during the C–P transition occurred earlier in western equatorial Pangea (Fig. 2) in the area roughly corresponding to the modern Colorado Plateau of southwestern North America.
Located within the hypothesized epicenter of dryland vertebrate expansion (Pardo et al., 2019), the Cutler Group in southeastern Utah, USA (Figs. 1–3), is ideal for studying this environmental and ecological transition. This unit's abundant fossil record (Fig. 1) and extensive outcrops of interbedded marine and continental “redbed” sediments preserve a diverse suite of depositional environments and associated biota spanning the C–P transition (Fig. 3), properties which make them a valuable resource for understanding the effects of this climatic transition on paleoequatorial ecosystems. Nevertheless, limited marine-biostratigraphic and geochronologic control (Fig. 3b) hinders a comprehensive paleoenvironmental reconstruction of these sediments and confident comparison to other contemporaneous fossil assemblages.
This collaborative research project, titled PERMIA (Paleozoic Equatorial Records of Melting Ice Ages), seeks to address these issues by studying the Elk Ridge no. 1 core (ER-1). This legacy core, collected in 1981 within what is now part of Bears Ears National Monument (Fig. 3a), recovered a significant portion of the lower Cutler Group and underlying units, making it an ideal archive for studying paleoenvironmental change during this critical period in terrestrial vertebrate evolution. Work is ongoing, yet much has been learned about the stratigraphic and geophysical properties through initial core processing. The purpose of this contribution is to introduce the project, share preliminary lithologic and geophysical results from the analysis of a legacy core (Elk Ridge no. 1), revise the position of lithostratigraphic boundaries within that core to align more accurately with the currently recognized regional stratigraphic framework, and discuss ongoing work with the core and complementary outcrop sites.
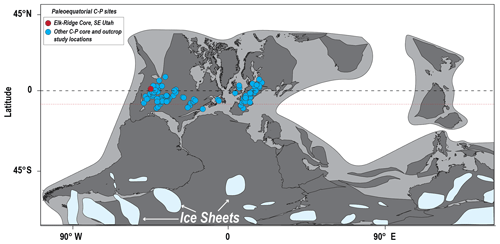
Figure 2Plate configuration of Pangea during the Late Carboniferous Period (Late Pennsylvanian), modified from Pardo et al. (2019). The red circle represents the paleogeographic location of our study area, including the Elk Ridge no. 1 core (ER-1). The light-blue circles represent other important paleoequatorial Late Carboniferous to Early Permian fossil sites (Pardo et al., 2019). Approximate paleogeographic locations and extents of the late Paleozoic ice sheets are modified from Isbell et al. (2012) and Kent and Muttoni (2020). The red dotted line shows the approximate position of the equator in the early Permian (Pardo et al., 2019), which is meant to show that many of the C–P localities would have been transported north of the Equator at this later time.
1.1 Motivation for studying the core
Few sites outside of the southwestern United States preserve a record complete enough to explore the relative contributions of paleoenvironmental change driven by global climate and continental drift in paleoequatorial C–P terrestrial ecosystems. Given the quality of its fossil record and expansive outcrops of shallow marine and continental rocks spanning the C–P transition, the canyon country of southeastern Utah is an ideal testing ground for these timely research questions (Reese, 2019; Gay et al., 2020). The rare juxtaposition of continental vertebrate-bearing fluvial and eolian redbeds interbedded with marine carbonate beds in a relatively continuous stratigraphic sequence makes southeastern Utah a unique window for studying synchronous, glacio-eustasy-driven oceanic and terrestrial events. In southern San Juan County, the lower Cutler Group include the fossiliferous terrestrial redbeds of the Halgaito Formation, which form the skirts and pedestals of the isolated buttes in Valley of the Gods (Fig. 3b) and the overlying eolian Cedar Mesa Sandstone. However, large portions of the Cutler Group in the region (including Valley of the Gods) are restricted to exposures along vertical cliff faces, rendering them inaccessible to outcrop-based, high-resolution paleoclimatic sampling.
The ER-1 core, collected ∼ 25 km north of Valley of the Gods (Fig. 3a), contains a relatively complete and minimally altered record of the Hermosa Group through the Cedar Mesa Sandstone, both of which were deposited in a position more proximal to the center of Paradox Basin (Fig. 4). The crucial paleogeographic position of ER-1, located further from the late Carboniferous uplift of the “Ancestral Rocky Mountains” (Fig. 4a) (Moore et al., 2008; Sweet et al., 2021), reduces tectonic autocyclic influences, thus enhancing the possible preservation of orbital climate signals. Furthermore, ER-1 exhibits a higher proportion of carbonate interlayers compared to southern outcrop sites (Figs. 3–4), offering a promising opportunity to utilize biostratigraphic methods for core dating. Therefore, ER-1 is one of the best opportunities to collect high-resolution paleoclimatic data from a nearly complete record of the C–P transition within the uppermost Hermosa Group (Honaker Trail Formation) and the overlying lower Cutler Group.
1.2 Geologic setting
In southern Utah's Paradox Basin, the C–P transition is encompassed by the lower portion of the Cutler Group (Scott, 2013; Huttenlocker et al., 2018), a ∼ 600 m thick succession of marginal-to-shallow marine and distal floodplain sediments deposited between rising salt anticlines (Condon, 1997). In the Colorado Plateau region, most early authors subdivided the Cutler Group into approximately four subunits, which they originally named the Halgaito tongue, Cedar Mesa Sandstone, Organ Rock tongue, and De Chelly Sandstone (Fig. 5) (Baker and Reeside Jr., 1929; Baker, 1936; Orkild, 1955; Sears, 1956; Baars, 1962; O'Sullivan, 1965). Wengerd (1958, 1963) revised the nomenclature, incorporating “Rico” as the basal-most unit and elevating Cutler and Halgaito to group and formation status, respectively. In the northern part of the basin, Baars (1962) raised the Organ Rock to formation status and considered lower Cutler-like units to be assignable to the Elephant Canyon Formation. In contrast, Loope et al. (1990) argued that all units below the Cedar Mesa Sandstone be redefined as the informal “lower Cutler beds” until regional correlations could be clarified, removing the Elephant Canyon Formation and calling into question the validity of strata assigned to Rico, given that its type section is located in western Colorado (Cross and Spencer, 1900). Nevertheless, the term “Halgaito” has continued to be used among researchers studying the Valley of the Gods and Mexican Hat region (Murphy, 1987; G. S. Soreghan et al., 2002; M. J. Soreghan et al., 2002; DiMichele et al., 2014; Golab, 2016; Huttenlocker et al., 2018, 2021; Golab et al., 2018; Tobey, 2020) (Fig. 5).
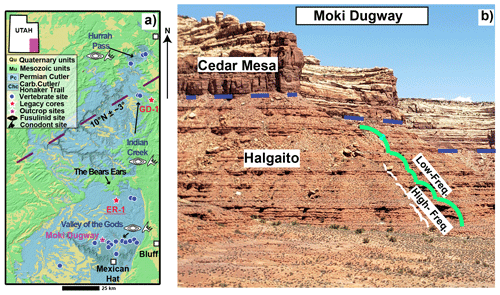
Figure 3(a) Map showing the extent of Cutler Group exposures in the study area, modified from Hintze et al. (2000) with paleolatitude estimates from van Hinsbergen et al. (2015) using the 300 Ma paleopole. Moki Dugway is represented with a purple star, while red stars designate the approximate locations of the Gibson Dome (GD-1) and Elk Ridge (ER-1) cores. Blue circles represent known vertebrate sites. Fossil sites with conodont and fusulinid occurrences are designated with their respective symbols. (b) Exposures of the Halgaito Formation (lower Cutler beds) and Cedar Mesa Sandstone at Moki Dugway; lithologic cycles in Halgaito are highlighted.
In the northwest–southeast-trending Paradox Basin, located on the western margin of the Pangean supercontinent (Fig. 4a–b), the Carboniferous–Permian units of the Cutler Group consist of three parallel and time-successive facies belts – the southwestern carbonate shelf, the northeastern siliciclastic wedge, and the mid-basin erg and evaporite belt (Fig. 4c). The group ultimately terminated with the expansion of coastal dune and loessite facies, indicative of pronounced seasonal aridity (Loope, 1984; Murphy, 1987; Condon, 1997; Doelling, 2002; Doelling et al., 2002; G. S. Soreghan et al., 2002; Dubiel et al., 2009; Jordan and Mountney, 2010, 2012; Scott, 2013; Golab et al., 2018; Tobey, 2020).
Near the Monument Upwarp, around the present-day Valley of the Gods, the Halgaito Formation represents a southern wedge of terrestrial redbeds that formed during the early phases of this aridity trend (Figs. 1, 3–5). Farther north, near the ER-1 core and the Bears Ears buttes (Fig. 3a), the basal Cutler unit consists of fluvial, aeolian, and shallow-marine facies informally known as the “lower Cutler beds” (Murphy, 1987; Condon, 1997). The Halgaito Formation intertongues with and is laterally equivalent to the lower Cutler beds (Figs. 4–5), both of which display evidence of progressively drier climates during the late Paleozoic (Murphy, 1987; Condon, 1997; G. S. Soreghan et al., 2002; Jordan and Mountney, 2010, 2012). This steady shift ultimately culminated in deposition of the overlying Cedar Mesa Sandstone, a set of thick, extensive, and predominantly eolian deposits overlying the lower Cutler beds throughout much of Paradox Basin, including the eponymous Cedar Mesa, greater Canyonlands area, and Indian Creek areas (Fig. 3a). By contrast, in the Hurrah Pass area southwest of Moab (Kane Creek Anticline), the Cutler Group only consists of the lower Cutler beds and an upper arkosic facies that is undivided but that may be equivalent to the Cedar Mesa and Organ Rock formations farther to the south and west (Billingsley and Huntoon, 1982; Langford and Chan, 1988; Condon, 1997; Carpenter and Ottinger, 2018).
Despite local variation, previous workers have observed that the strata across these areas show pronounced cyclicity (Doelling, 2002; G. S. Soreghan et al., 2002; Jordan and Mountney, 2010, 2012). Within the Halgaito Formation, these lithologic cycles occur as alternating sequences of loessite–paleosol couplets (Murphy, 1987) with accompanying cyclicities observed in rock magnetic properties (G. S. Soreghan et al., 2002), whereas in the overlying Cedar Mesa Sandstone they consist of alternating dune–interdune couplets (Mountney and Jagger, 2004). Traditionally, the cyclic nature of the Cutler Group has been speculated to record long eccentricity Milankovitch cycles (G. S. Soreghan et al., 2002; Jordan and Mountney, 2012), whereas the larger-scale transition from the fluvial or marine lower Cutler beds to the eolian Cedar Mesa Sandstone is commonly interpreted as representing a longer-term desertification event of ambiguous origin (Soreghan and Soreghan, 2007; Soreghan et al., 2008, 2020, 2023; Tabor and Poulsen, 2008).
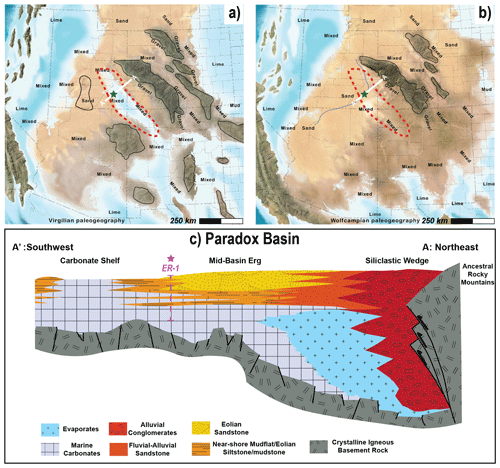
Figure 4(a) and (b): paleogeography of Paradox Basin (red outline) during the Virgilian and Wolfcampaian, respectively (modified from Blakey, 2019); green stars are the locations of ER-1. (c) Southwest–northeast cross section of Paradox Basin during the C–P. Note the three successive facies of the Cutler Group: siliciclastic wedge, mid-basin erg, and carbonate shelf. The vertical green dashed line to the left represents ER-1. Figure 4c is modified from Baars and Stevenson (1981), Goldhammer et al. (1991), Guthrie and Bohacs (2009), and Whidden et al. (2014). The location of line A–A' is shown in Fig. 4a–b.
Evidence for large-scale aridification during the Carboniferous–Permian is not restricted to Paradox Basin; indeed, coeval basins throughout Pangea all display a decline in lithologic characteristics associated with wet climates (i.e., coal, laterite, and bauxites) and an increase in those that indicate arid climates (calcrete, evaporites, and eolianites) (Soreghan and Soreghan, 2007; Soreghan et al., 2008, 2020, 2023; Tabor and Poulsen, 2008; Pardo et al., 2019). This environmental shift is also reflected in paleoequatorial fossil sites (blue circles in Fig. 2), which show a shift to more abundant terrestrial taxa in the early Permian (Pardo et al., 2019). Due to the widespread nature of central Pangean arid lithologies and terrestrial life during the Late Carboniferous–Cisuralian, it is not unreasonable to postulate that the aridity trend exhibited by the lower Cutler Group is related to a near-global climatic shift as opposed to an autocyclic event or lateral migration of facies independent of climate. Nevertheless, uncertainty persists with regards to inter-basinal temporal correlation between the Cutler Group and other C–P assemblages, such as the classically studied mid-continental and Texas–Oklahoma regions in North America, the Dunkard Group in West Virginia and Pennsylvania, the Lodève Basin in France, and the Saar–Nahe Basin in Germany (blue circles in Fig. 2) (Romer, 1945; Parrish, 1978; Boy and Fichter, 1982; Craddock et al., 1989; Eberth et al., 2000; Uhl et al., 2004; Tabor and Poulsen, 2008; Michel et al., 2015; Pfeifer et al., 2020). Therefore, one of the goals of PERMIA is to analyze the ER-1 core with the goal of building a robust dataset of biostratigraphic and geochronological age controls and paleoclimate proxies with unambiguous superposition, allowing for the correlation between the lower Cutler Group and these other late Paleozoic records. Additionally, this work complements the ongoing Deep Dust Project targeting mid-continent and European sites recording the Early to Middle Permian collapse of the Late Paleozoic Ice Age (blue circles in Fig. 2) (Soreghan et al., 2020).
2.1 Historical context and selection by PERMIA
The ER-1 core was collected in the early 1980s as part of a national study for determining potential subsurface storage sites for high-level radioactive waste. This federal project, named the National Waste Terminal Storage Program, was founded in 1976 by the Energy Research and Development Administration (ERDA), a precursor to the United States Department of Energy (DOE) (Woodward-Clyde Consultants, 1982b). This initiative aimed to identify suitable locations for the permanent, long-term disposal of high-level radioactive waste arising from nuclear weapons production and electricity generation (Interagency Review Group on Nuclear Waste Management, 1978; Woodward-Clyde Consultants, 1982a, b; Thackston et al., 1984). Due to the long half-life of radioactive waste, ideal long-term geologic storage necessitates that the toxic materials be relocated to tectonically inactive areas where they will be completely sealed and isolated from adjacent hydrologic units over geologic timescales (i.e., ∼ 100 000 years) (European Commission, 2004; Ahn and Apted, 2010). At the time, subsurface salt deposits, which are highly impermeable, were considered one of the best candidates for deep geologic repositories (Pierce and Rich, 1962; Hansen and Leigh, 2011). This led to the investigation of several evaporite-bearing basins in the continental United States, including Paradox Basin in southern Utah (Fig. 4). To better understand both the properties of the Paradox evaporites and the overlying hydrogeologic system, at least two rock cores were collected by Woodward-Clyde Consultants, the ER-1 and Gibson Dome no. 1 (GD-1) cores (Fig. 3a) (Woodward-Clyde Consultants, 1982a, b; Thackston et al., 1984). After several years of investigation, the Elk Ridge site was abandoned in favor of the Yucca Mountain, Nevada, site (Blanchard, 1985; U.S. Department of Energy, 1986; Keeney, 1987; Merkhofer and Keeney, 1987; McCleary, 1989; Stuckless and Levich, 2016), but the ER-1 and GD-1 cores were archived at the Austin Core Research Center and have become available for scientific study.
For the PERMIA project, the decision to prioritize the ER-1 core over the GD-1 core was primarily influenced by the need to minimize the potential influence of salt tectonics. Despite their shared penetration of formations of interest and biostratigraphic controls, GD-1's proximity to regions characterized by significant salt-tectonic activity (Fig. 4) raised concerns about potential alteration to the sedimentary record. In contrast, ER-1 is situated in a more geologically stable area, making it the most favorable choice for conducting precise paleoclimatic analyses and preserving the integrity of the sedimentary data required for PERMIA. In addition, the ER-1 site is more centrally located between rich outcrop archives of paleontological and paleoenvironmental information that are also part of our project, between the Valley of the Gods–Mexican Hat area to the southwest and the Indian Creek and greater Canyonlands areas to the north.
2.2 Drilling, initial handling, and storage of ER-1
The ER-1 core is a continuous 933.5 m long 6.35 cm diameter (HQ) core which was collected by operators Woodward-Clyde Consultants from San Juan County, Utah, USA (Fig. 3), between 5 August and 30 September 1981. Woodward-Clyde Consultants contracted Zimmerman Well Service for the drilling and installation of a 127.7 m (419 ft) surface casing, which was prepared using an 8 ton well drilling rig. Woodward-Clyde Consultants then contracted Boyles Brothers for coring operations, employing a Chicago-Pneumatic Model 50 rig (additional details available in Woodward-Clyde Consultants, 1982a).
The core was collected from an initial depth of 127.7 m (419 ft) to a final depth of 1061.2 m (3481.6 ft). To prevent dissolution of Paradox Formation evaporites, the drilling fluid consisted of a cellulose polymer mixed with “Moab brine”, a salt-saturated fluid produced by pumping freshwater into the Paradox Formation at Moab, Utah (Woodward-Clyde Consultants, 1982a; Thackston et al., 1984). The cellulose polymer, specified in Woodward-Clyde (1982a) as Mark IV and Hi-Vis, was provided by Western Mud Company (Woodward-Clyde Consultants, 1982a), which is now known as GEO Drilling Fluids, Inc (GEO Drilling Fluids Inc., 2023). Unfortunately, during the drilling process, substantial amounts of lost circulation were encountered while drilling through the Middle Pennsylvanian Pinkerton Trail Formation at ∼ 1061 m depth, preventing the penetration of the underlying Mississippian Leadville Limestone as originally planned (Thackston et al., 1984; Woodward-Clyde Consultants, 1982a). Nevertheless, the successful collection of the Carboniferous–Permian lower Cutler Group, which comprises the top ∼ 313 m of the ER-1 core, makes it ideal for accomplishing the research objectives of PERMIA. Geologic mapping of the area indicates that beds have a regional dip of no more than 1–3 ° (Lewis et al., 2011). This, in conjunction with the minimally reported borehole deviation (Woodward-Clyde, 1982a), suggests that the cores represent a near-vertical cross section of the rock formations under study.
Detailed descriptions of core collection and handling are provided in Woodward-Clyde (1982a). To briefly summarize, the wire-line diamond coring method and split inner-core barrels were utilized to minimize damage to the core. Core runs, measured by Woodward-Clyde in feet, were continuously collected at ∼ 10 ft (∼ 3.05 m) intervals. Once brought to the surface, core runs were transported to an on-site core handling building, where they were opened, placed onto roller tracks, fitted together at breaks, examined for core loss, washed, measured, and directly marked with depth measurements (in feet) at 1 ft (∼ 30.5 cm) intervals. Subsections of individual core runs were completely sampled on the occasion that said portions exhibited notable amounts of hydrocarbons. Otherwise, the core runs were cut into 2 ft (∼ 61 cm) sections with a diamond saw, wrapped in plastic, and placed into cardboard core boxes. Depth measurements, run numbers, locations, dates, and, if present, core loss and/or complete removal of sampled subsections were noted directly on the box lid as well as on labels taped to the box bottom.
The cores were shipped to an interim storage facility known as the “ONWI core storage facility”, which was in Lakewood, Colorado (Woodward-Clyde, 1982a). The cores were eventually transferred to the Austin Core Research Center (CRC), part of the Bureau of Economic Geology in Austin, Texas. As of 2022, 98.3 % of the top 451.1 m of ER-1 remained in storage at the CRC. This exceptional level of preservation can be attributed to a combination of factors, including a high initial drilling recovery rate of 99.8 % (450.3 m recovered out of 451.1 m drilled) and a relatively minor amount of sampling from previous studies, totaling approximately 6.98 m, or 1.5 %, of removal of whole-core (unsplit) samples.
2.3 Previous research
2.3.1 Geologic descriptions from Woodward-Clyde
Originally described by Woodward-Clyde (1982b), the ER-1 core recovered ∼ 933.5 m of late Paleozoic sediment, encompassing the lower portion of the Cedar Mesa Sandstone down to the Middle Pennsylvanian Pinkerton Trail Formation. The incomplete recovery of the Cedar Mesa Sandstone is a result of the ER-1 borehole being drilled and cased to a depth of 127.7 m (419 ft) prior to coring. This interval included Cenozoic surficial sediments directly overlying exposures of the upper portion of the Cedar Mesa Sandstone. Therefore, though the original report measured the total thickness of the Cedar Mesa Sandstone at the drilling site at 252.98 m, the ER-1 core only recovered the lowermost 128.3 m of this unit.
In the original lithologic description, the Cedar Mesa Sandstone is generally described as a sequence of light-colored, well-sorted, quartz-rich, carbonate-cemented, and primarily cross-bedded sandstones. Though primarily comprising sandstone, a limited number of thin reddish siltstone and sandy siltstone interbeds were also observed. Below the Cedar Mesa Sandstone is a heterogeneous collection of siltstone, sandy siltstone, and sandstone with occasional limestone interbeds. Measured to a thickness of 138.9 m, Woodward-Clyde (1982b) referred to this section as the “Elephant Canyon Formation”, a now obsolete name roughly equivalent to what are currently referred to as the “lower Cutler beds” (Loope et al., 1990; Jordan and Mountney, 2012).
Underlying the Elephant Canyon Formation is the Upper Carboniferous Hermosa Group, which consists of the Honaker Trail, Paradox, and Pinkerton Trail formations in descending order. In ER-1, the Honaker Trail Formation was measured to a thickness of ∼ 374.3 m and primarily consists of calcite-rich carbonate rocks (primarily limestones and cherty limestones). Nevertheless, ∼ 30 % of the Honaker Trail in ER-1 is described as consisting of siliciclastics (siltstones and sandstones) and dolomitic interbeds. Woodward-Clyde noted a gradational contact between the Honaker Trail and the underlying Paradox Formation, the latter of which was measured to a thickness of 291.4 m; 29 % of the Paradox Formation in ER-1 is described as halite, with the remainder consisting of siltstone, shale, anhydrite, limestone, and dolomite. Woodward-Clyde described this unit as highly cyclical with four to six sequences correlated with the regionally recognized salt cycles of the Paradox Formation (specifically, cycles 6, 7, 9, and 13 and potentially cycles 15 and 16) (Peterson and Hite, 1969; Hite and Buckner, 1981; Woodward-Clyde Consultants, 1982a, b). The lowest unit of the Hermosa Group and the deepest deposit recovered by ER-1 is the Pinkerton Trail Formation. Woodward-Clyde (1982b) describes the formation as primarily consisting of limestone and calcareous siltstone, with some minor units of dolomite and anhydrite. Though drilling plans originally intended to recover both the underlying Molas and Leadville formations, substantial drilling fluid loss forced the termination of drilling activities after recovering only 51.8 m of the Pinkerton Trail (Woodward-Clyde Consultants, 1982a, b).
2.3.2 Previous age control
Since recovery, various researchers have examined portions of the ER-1 core for biostratigraphic analysis, sedimentological analysis, and regional stratigraphic correlations (Loope and Watkins, 1989; Atchley, 1990; Nail et al., 1994, 1996; Tromp, 1995; Raup and Hite, 1996; Tuttle et al., 1996; Nail, 1996; Atchley and Loope, 1993; Petrychenko et al., 2012; Rasmussen and Rasmussen, 2018). The identification of Late Carboniferous conodonts and fusulinids within ER-1 by Nail et al. (1996) implies a late Missourian through middle Virgilian age for the Honaker Trail Formation and a late Virgilian age for the lowermost limestones of the lower part of the lower Cutler beds (Fig. 7). These age constraints are consistent with outcrop work in nearby Arch Canyon and Valley of the Gods, where Huttenlocker et al. (2021) identified the conodont Adetognathus sp. B in the upper part of the lower Cutler beds. The presence of this conodont species, now referred to as Adetognathus carlinensis (see Beauchamp et al., 2022), which is known from the C–P interval (latest Gzhelian–early Asselian) of the Canadian Arctic and eastern–central British Columbia (Henderson et al., 1995), suggests that the C–P transition can be identified in the lower Cutler beds, including the ER-1 site. To this end, see the “Continuing science plans” section of this paper for ongoing research on the biostratigraphy of the ER-1 core.
In early 2023, the top 450 m (corresponding to a depth of 127.77–581 m) of the ER-1 core was transported from the Austin Core Research Center to the Continental Scientific Drilling Facility at the University of Minnesota for preparation and analysis. Upon arrival, all core sections were photographed in their respective boxes and placed in transparent plastic core liners with end-caps indicating the stratigraphic top and bottom of each core section. The liners enabled detailed scanning and processing workflows aligned with best practices established in numerous recent scientific drilling projects (e.g., Clyde et al., 2013; Cohen et al., 2016; Olsen et al., 2018). Evidence of core degradation was minimal, limited to salt crystals from dried drilling fluid on the surface of some sandstone core segments. The geophysical properties of the whole cores were measured using a Geotek Standard Multisensor Core Logger and included magnetic susceptibility (loop), gamma density, p-wave velocity and amplitude, natural gamma radiation, and electrical resistivity.
Using a diamond-blade rock saw with continuous deionized water flush, the core was split lengthwise into two halves: an archive half which was placed into storage and a work half which was subjected to further analysis and subsampling. To eliminate saw marks and enhance the clarity of the split core face, the working halves were polished using either dry or wet rock polishers. This step was necessary to more clearly image sedimentary structures and microfossils that were obscured by the cutting process. The polished working halves were imaged at 20 pixels per millimeter resolution using the Geotek Core Imaging System. Additionally, high-resolution point magnetic susceptibility and color reflectance spectrophotometry of the core face were measured using a Geotek XYZ Multisensor Core Logger. Core depth scales were generated by applying a linear compression factor for core runs to fit the drilled interval when recovery wad > 100 % (equivalent to the CSF-B depth scale used in scientific ocean drilling, e.g., Expedition 337 Scientists, 2013).
Employing Corelyzer software, the PERMIA team directly compared the physical core sections with their high-resolution images and accompanying geophysical datasets (Ito et al., 2023). These visual representations enabled comprehensive lithologic descriptions of each core section, which were recorded using the PSICAT software program (Reed et al., 2007).
One of the initial goals of PERMIA was to refine the stratigraphy of ER-1 within an updated regional stratigraphic framework using currently accepted terms (Loope et al., 1990; Fig. 5). Examination of the polished split cores and high-resolution core images (e.g., Fig. 6) in the stratigraphic context using the Corelyzer visualization environment (Ito et al., 2023) allowed for more accurate determinations of lithology, stratigraphic contacts, sedimentary structures, and microfossils. By comparing this new dataset to recent stratigraphic work (Loope et al., 1990; Dubiel et al., 1996, 2009; Condon, 1997; Ritter et al., 2002; Mountney and Jagger, 2004; Jordan and Mountney, 2012), PERMIA updated the stratigraphy of the upper core to reflect more accurately the accepted lithostratigraphic nomenclature (Fig. 5) and associated boundaries (Fig. 7). Specifically, we place the Cedar Mesa Sandstone–lower Cutler beds contact at 318.9 m depth and the lower Cutler beds–Honaker Trail Formation (Cutler Group–Hermosa Group) boundary at 442.6 m depth. These placements are consistent with the lithostratigraphic boundaries as observed in nearby outcrops (e.g., the Arch Canyon and Valley of the Gods area) and descriptions in the published literature.
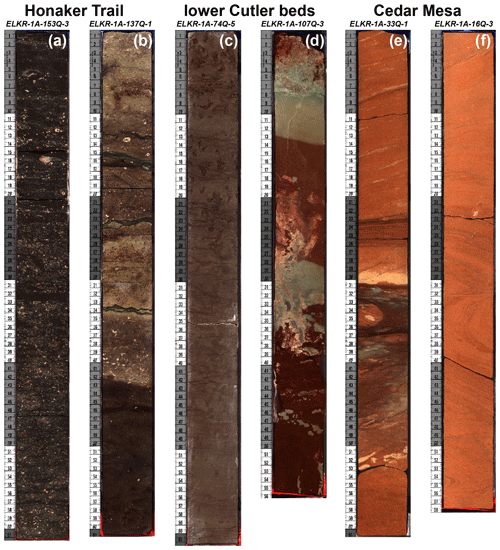
Figure 6Representative facies in core segments from the ER-1 core. (a) Fossiliferous limestone layer from the Honaker Trail Formation. (b) Contact between a carbonaceous siltstone (lower darker unit) and a lighter-colored fossiliferous limestone unit (upper light-grey unit) in the Honaker Trail Formation. (c) Calcareous siltstone with abundant burrows in the lower Cutler beds. (d) Siltstone redbed units in the lower Cutler beds, with abundant reduction zones. (e) Cross-bedded sandstone unit from the Cedar Mesa Sandstone with a dark-brown siltstone interbed. (f) Cross-bedded sandstone unit from the Cedar Mesa Sandstone.
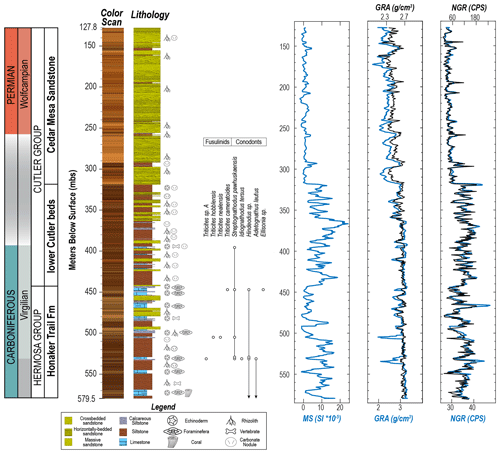
Figure 7Generalized stratigraphic column of the top 450 m of the ER-1 core. Left to right: color profile from spectrophotometry, lithology, published biostratigraphic controls (from Nail et al., 1996), core magnetic susceptibility (MS), gamma ray attenuation density (GRA) from both core (blue) and well log (black), and natural gamma radiation from both core (blue) and well log (black). Though all three lithologic units are present throughout the core, limestones, siltstones, and sandstones are thickest and most common within the Honaker Trail, lower Cutler beds, and Cedar Mesa formations, respectively. Moreover, there is a notable negative secular trend in both the magnetic susceptibility and gamma density values as the lower Cutler beds transition into the Cedar Mesa Sandstone. Note that the geologic timescale uses the regional terms Virgilian and Wolfcampian, which roughly correspond to the Gzhelian and Asselian stages, respectively. Grey zones between chronostratigraphic terms indicate uncertainty in boundary placement.
Our placement of the Cutler–Honaker Trail boundary is at a marked change to redder, predominantly non-calcareous siltstone with less abundant and much thinner marine carbonate layers and more common sandstone layers. This is consistent with the lithologic changes observed in outcrops along the southern margin of Cedar Mesa (e.g., Valley of the Gods and Goosenecks of the San Juan State Park areas), where a mixed siliciclastic–carbonate succession with abundant marine limestones (the Honaker Trail Formation) is overlain by siltstone and sandstone redbeds with rare, thin marine limestones (the local equivalent of the lower Cutler beds called the Halgaito Formation) (Baker, 1936; G. S. Soreghan et al., 2002; Ritter et al, 2002; Huttenlocker et al., 2021). The color change associated with this boundary is apparent in the core line scan image stack (Fig. 7), where our boundary placement also marks a change from drab brown and tan colors below to darker reddish-brown colors above. Also notable is that our placement of the Honaker Trail–Cutler boundary is just above three sandstone units associated with marine limestones (sandstones otherwise being rare in the Honaker Trail Formation), a lithologic association also observed in outcrops in the uppermost Honaker Trail Formation at its eponymous locality just south of Cedar Mesa (Ritter et al., 2002: Figs. 3, 5). Some previous stratigraphic studies emphasize that the contact in this area forms a change in the weathering profile from the canyon or cliff down-section to the slope and pillar-forming lower Cutler above (e.g., Baker, 1936; Gregory, 1938), but this cannot be evaluated in the core.
We place the lower Cutler beds–Cedar Mesa Sandstone boundary at the base of the first thick (> 20 m) cross-bedded sandstone, marking a rather abrupt change from strata dominated by dark reddish-brown siltstone with less common thin (typically < 5 m thick) sandstone packages, to thick (> 10 m), predominately cross-bedded light-colored sandstones separated by occasional thin reddish-brown siltstones (all < 5 m thick and often less than 1 m thick). This boundary placement is just above the highest unambiguous marine unit, a wavy-laminated dark reddish-brown sandy siltstone between 323 and 318.9 m depth containing abundant disarticulated echinoderm elements. Locating the lower Cutler–Cedar Mesa boundary above the last marine unit and at the base of the first thick sandstone sequence is also consistent with its placement in outcrops by recent stratigraphic studies (e.g., Stanesco and Campbell, 1989; Loope et al., 1990; Dubiel et al., 1996, 2009; Jordan and Mountney, 2012; Huttenlocker et al., 2021; Pettigrew et al., 2021). Our boundary placement is also associated with a noticeable decrease in magnetic susceptibility, gamma density, and natural gamma radiation values (Fig. 7), which other authors have observed at this contact from wells in the same area (Fig. 8 in Condon, 1997).
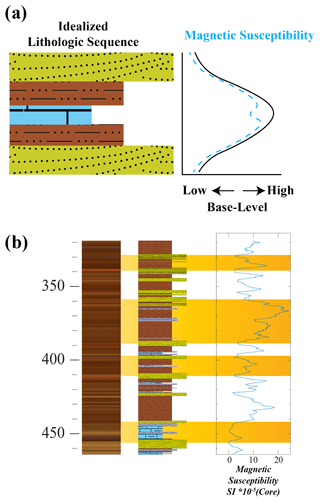
Figure 8(a) Idealized lithologic sequence and associated response in MS. In this model lower base levels correspond to more arid conditions, resulting in sandstones composed of relatively weakly magnetic to non-magnetic minerals (i.e., hematite). As base levels rise, conditions become wetter, corresponding to finer and more magnetic minerals. However, fully marine environments (limestone) correspond to a magnetic decrease. As the base level lowers and arid conditions return, sandstones composed of weak magnetic material are deposited once again. (b) ER-1 from 460 to 320 m CSF-B, with certain cycles highlighted in orange. Note that each cycle is book-ended by sandstone layers.
Overall, the core is dominated by a relatively small number of lithologies. Carbonate layers are typically either sandy to silty limestones containing abundant marine fossils (echinoderms, brachiopods, bivalves, fusulinids) or thin carbonate layers devoid of visible fossils and with mostly nodular textures. The fossiliferous marine carbonate facies are only observed in the Honaker Trail Formation and lower Cutler beds, whereas the non-fossiliferous carbonates are found throughout the core and appear to be mostly non-marine in origin (i.e., they are associated with pedogenically modified siltstones). Carbonate-rich siltstones are often associated with both of these limestone facies and are also present as thin layers associated with dark reddish-brown siltstones within the Cedar Mesa Sandstone. Brown to dark reddish-brown siltstones and sandy siltstones are either parallel-bedded and are sometimes laminated or massive. The latter are often both mottled and contain carbonate nodules. These siltstone facies comprise the majority of the Honaker Trail Formation and lower Cutler beds but also form thin (< 5 m thick) layers between the thick sandstones of the Cedar Mesa Sandstone. Predominantly orange, tan, and pinkish-grey fine- to medium-grained sandstones are typically trough cross-bedded or parallel-bedded, though a small number of units are apparently massive (acknowledging the restricted lateral view of a core). Sandstones are rare in the Honaker Trail Formation, except for an interval between 440 and 500 m depth, more common (but still subordinate to siltstone) in the lower Cutler beds, and dominate the Cedar Mesa Sandstone. In the latter, they are mostly trough cross-bedded, but parallel-bedded and massive units are present, particularly just above and below silty layers. The fact that we often observe gradational transitions between lithologies (i.e., sandy siltstones between sandstones and siltstones, calcareous siltstones between carbonates and siltstones) and a lack of observable erosive contacts supports our inference that the core lacks any significant discontinuities.
This variety of lithologies can be grouped into three informal lithofacies associations: (1) clastic to silty fossiliferous limestones; (2) massive to horizontally bedded calcareous and non-calcareous siltstone; and (3) massive, horizontally bedded, and cross-bedded fine-grained sandstone (Figs. 6–7). Siltstones in all three formations show extensive evidence of pedogenic modification (e.g., mottling, carbonate nodules) (Fig. 6). Though all three rock types are present within each formation, limestones become less abundant up-section, being most common and thickest in the Honaker Trail Formation, siltstones are most common in the Honaker Trail Formation and lower Cutler beds, whereas cross-bedded sandstones dominate the Cedar Mesa Sandstone.
Cyclic variation both within and among these three rock types is common throughout the core, regardless of stratigraphic unit. In the Honaker Trail Formation, this cyclicity is manifested predominantly as siltstone–limestone couplets, whereas in the overlying lower Cutler beds it consists of siltstone–sandstone couplets, with occasional limestone or calcareous siltstone layers in the middle of the siltstones. In the Cedar Mesa Sandstone, the main cyclicity is an alternation between trough cross-bedded sandstone, parallel-bedded or massive sandstone, and thin siltstone beds. These lithologic cycles match those observed by other authors in equivalent outcrops in Paradox Basin (e.g., Ritter et al., 2002; Mountney, 2006; Williams, 2009; Jordan and Mountney, 2012; Langford and Salsman, 2014; Olivier et al., 2023). Comparing these lithologic cycles to the geophysical data, siltstones generally have a much higher magnetic susceptibility than either sandstone or limestone layers. This results in a distinct cyclical pattern in the magnetic susceptibility data (Figs. 7–8), which aligns with corresponding cycles in the lithostratigraphy. On a larger scale, the magnetic data exhibit a negative secular trend as the lower Cutler beds transition into the more sandstone-dominated Cedar Mesa Sandstone (Fig. 7).
With initial core processing completed, the micropaleontological, geochemical, and paleomagnetic properties of ER-1 are currently being analyzed, the primary goal being the construction of a robust paleoenvironmental reconstruction anchored by reliable geochronologic controls. Though no known volcanic ash layers (and few juvenile detrital zircons) have yet to be identified in the lower Cutler Group, several approaches are available to date the core effectively. First, Nail et al. (1996) already recovered biostratigraphically useful fusulinids and conodonts from several levels within the core, and polished split core segments of ER-1 revealed highly fossiliferous carbonate layers in the Honaker Trail Formation (Fig. 6) and the lower Cutler Group (Fig. 7); together, this implies that the core can be precisely dated using biostratigraphic methods. Such an approach has been applied successfully to the underlying Carboniferous Hermosa Group (Ritter et al., 2002) as well as outcrop exposures of the lower Cutler beds in the region, where widespread presence of conodonts in marine carbonate layers (Figs. 3, 4) allowed for correlation with well-resolved marine biochronologies from elsewhere (Fig. 7) (Huttenlocker et al., 2021). Indeed, conodont biostratigraphy is the gold standard used to define the C–P boundary at both the Asselian GSSP (Usolka, Ural Mountains) (Chernykh and Ritter, 1997; Chernykh et al., 1997) and North American mid-continental sections (Ritter, 1995; Wardlaw, 2005; Boardman et al., 2009; Wardlaw and Nestell, 2014; Henderson, 2018). Formal identification of the C–P boundary within the Cutler Group remains critical for correlating Utah's rock record with the geologic timescale as well as other relevant C–P paleoenvironmental and paleontological archives across Pangea. Complementary to the biostratigraphic approach, the global marine record – a proxy for Phanerozoic seawater Sr (Veizer, 1989; McArthur et al., 2020) – shows a steep decrease from base-Ghzelian (latest Carboniferous) values of 0.7081–0.7087 to 0.7079–0.7081 by the end of the Asselian (earliest Permian) (Korte et al., 2006; Korte and Ullmann, 2018; Chen et al., 2018). Thus, a time series of isotope values from marine carbonates and the associated conodont bioapatite should accurately place the Cutler sequence along the C–P strontium reference curve. Therefore, we sampled all major marine limestone units in the studied portion of the core for conodonts, fusulinids, and isotopes.
Previous attempts at dating through paleomagnetic methods confirmed that deposition of the Cutler Group coincides with the Kiaman-reversed superchron (Gose and Helsley, 1972; Scott, 1975) (Fig. 1). This interval should also include the short-lived “Kartamyshian event”, a normal polarity magnetozone dated to ∼ 299 Ma (Fig. 1), and if found within this core and outcrop, it would serve as a useful maximum age constraint and datum for the top of the Carboniferous (Hounslow and Balabanov, 2016). Previous work (Gose and Helsley, 1972; Scott, 1975) indicates that the lower Cutler beds preserve a primary magnetic remanence, and we therefore should be able to identify the Kartamyshian event if it is present.
Furthermore, cyclic sedimentation patterns established in the Cutler Group (G. S. Soreghan et al., 2002; Jordan and Mountney, 2012; Golab, 2016; Golab et al., 2018; Tobey, 2020) strongly suggest that astronomically tuned geochemical and magnetic cyclostratigraphy can provide high-resolution age models for these deposits. Various studies have shown that long-term eccentricity cycles (∼ 405–413 kyr) have been stable throughout Earth's history (Olsen and Kent, 1999; Horton et al., 2012; Kent et al., 2017, 2018; Lantink et al., 2019; Olsen et al., 2019). Indeed, in the Canyonlands area, Jordan and Mountney (2012) identified lithologic cycles in the lower Cutler beds, which they hypothesized were reflective of long eccentricity cycles. The magnetic susceptibility record of ER-1 (Figs. 7–8), along with previous environmental–magnetic studies of the Halgaito Formation near Mexican Hat (G. S. Soreghan et al., 2002), demonstrate that cyclic facies changes correspond to variations in magnetic properties (Fig. 8). This provides strong evidence that it is possible to construct a magnetic cyclostratigraphic framework anchored by biostratigraphic, strontium isotope, and paleomagnetic age constraints.
This high-resolution geochronological dataset will be constructed in tandem with the measurement of various geophysical and geochemical paleoclimatic proxies. This will include the whole-core geophysical data presented in this paper (e.g., MS, NGR, color scans) (Fig. 7), high-resolution XRF core scans (in progress), and environmental magnetic (Verosub and Roberts, 1995; Evans and Heller, 2003; Liu et al., 2012) measurements of discrete specimens. Furthermore, similar datasets will be collected from outcrop sites (Fig. 3a), enabling the development of a robust and temporally constrained paleoenvironmental framework for this region during the C–P transition. Finally, this model will be correlated with a high-resolution dataset of georeferenced vertebrate sites and specimens (in progress) as a means of testing the Vaughn–Olson model (Pardo et al., 2019) and other important questions regarding the C–P transition.
-
Do independent paleoenvironmental proxies and magnetic mineral assemblages record a secular drying trend across the lower Cutler bed–Cedar Mesa Sandstone transition?
-
Is this transition rapid and concurrent with the established LPIA maximum (Gzhelian), or does it instead follow a longer-term trend that matches the paleomagnetically determined northward drift of North America into a more arid paleolatitudinal climate belt?
-
What effect, if any, did these paleoclimatic changes have on the evolution of C–P vertebrate communities?
Our analysis of the ER-1 core will improve the geochronological and paleoenvironmental context for the important paleontological and geological archives of the C–P transition preserved within Bears Ears National Monument. Thus, these core data enrich and elevate the global importance of the at-risk scientific resources preserved within the monument (Gay et al., 2020). By clarifying the processes driving equatorial climate and biotic change during the Late Paleozoic Ice Age, the study of the ER-1 core strengthens our ability to use the LPIA and its decline as a natural deep time analog that can be compared with ongoing anthropogenically driven global warming and ecological change. Data and conclusions from our ER-1 studies will be integrated into all levels of education and public engagement, from informal settings such as public exhibits in local communities in southeastern Utah to K-12 public school and higher-education classrooms. We are working with education experts at the Natural History Museum of Utah to develop curriculum resources, and the core images and associated data will be investigated and analyzed by undergraduate students, as one of us (RBI) has done with similar data from the CPCP project (Olsen et al., 2018).
By continuing to analyze the ER-1 core, we expect to gain further insight into the precise timing of regional late Paleozoic drying and its relationship with the collapse of the LPIA, ca. 304–280 million years ago. Furthermore, by correlating this new dataset with planned paleontological field work, we intend to enhance our comprehension of the biotic impacts resulting from Earth's last pre-Quaternary icehouse–hothouse state change. As present-day anthropogenic climate change threatens to destabilize a multitude of environments, improvements in our understanding of how major climate processes have impacted biomes in the past are increasingly relevant. To this end, the outcome of this study will benefit climate modelers, policy makers, ecologists, and the many communities impacted by climate change.
The archive and work halves of the ER-1 cores are permanently stored at the Austin Core Research Center (CRC), run by the Bureau of Economic Geology (BEG) in Austin, Texas.
JMGS, JMF, AKH, RBI, DR, RD, JM, KBS, RO, AS, and PS helped process the core, conduct measurements, and collect images. JMGS drafted Figs. 2, 4, 5, 6 and 7. AKH drafted Figs. 1 and 4. RBI and JMGS co-drafted Fig. 3. JMF and JMGS co-drafted Fig. 8. JMGS, JMF, AKH, RBI, CMH, MTR, and AN contributed to editing the final versions of the figures. All the authors have read and approved the current version of the manuscript and figures.
The contact author has declared that none of the authors has any competing interests.
Publisher's note: Copernicus Publications remains neutral with regard to jurisdictional claims made in the text, published maps, institutional affiliations, or any other geographical representation in this paper. While Copernicus Publications makes every effort to include appropriate place names, the final responsibility lies with the authors.
The authors thank the personnel at the CSD facility for their support. The authors would also like to thank the UT-Austin Bureau of Economic Geology – Core Research Center for providing access to the core. We are grateful to Don Rasmussen for originally suggesting we examine the ER-1 core. We would also like to thank Carolyn Bishoff and the library staff at Interlibrary Loan (ILL) at the University of Minnesota for acquiring the various reports by Woodward-Clyde Consultants (1982a, b). We are grateful to Judith Totman Parrish, an anonymous reviewer, and editor Ulrich Harms for comments that significantly improved the quality of the manuscript.
The University of Minnesota is built on the ancestral lands of the Wahpekute band that was ceded to the United States by the Treaty of Traverse des Sioux in July of 1851, in an agreement that was not paid in full and whose underlying aim was the dissolution of the Dakota culture. The university has also benefited from Chippewa and Dakota (Mdewakanton, Wahpekute, Wahpeton, and Sisseton bands) land ceded by treaty and given to the University of Minnesota via the Morrill Act. Due to its land-grant status, the infrastructure, financial foundations, faculty, students, and staff at the University of Minnesota all continue to benefit directly from these ceded lands, and we wish to acknowledge this support in our research.
This research has been supported by the National Science Foundation Division of Earth Sciences (grant nos. NSF-2221050, NSF-2219902, NSF-2219947, NSF-2153786, and NSF-1951112) and the College of Science and Engineering, University of Minnesota (grant no. UMN GIA-#500130).
This paper was edited by Ulrich Harms and reviewed by Judith Totman Parrish and one anonymous referee.
Ahn, J. and Apted, M. J.: Geological repository systems for safe disposal of spent nuclear fuels and radioactive waste, Woodhead Publishing, 792 pp., First Edition, https://doi.org/10.1533/9781845699789, 2010.
Atchley, S. C.: Influence of lowstand eolian erosional processes on stratigraphic completeness: sequence stratigraphy of the upper member of the Hermosa Formation (Upper Pennsylvanian), Paradox Basin, southeast Utah, Dissertation, University of Nebraska, Lincoln, Nebraska, 1–135 pp., ISBN 979-8-207-75988-3, 1990.
Atchley, S. C. and Loope, D. B.: Low-stand aeolian influence on stratigraphic completeness: upper member of the Hermosa Formation (latest Carboniferous), southeast Utah, USA, Special Publications of the International Association of Sedimentologists, 16, 127–149, https://doi.org/10.1002/9781444303971.ch9, 1993.
Baars, D. L.: Permian System of Colorado Plateau, Am. Assoc. Pet. Geol. Bull., 46, 149–218, https://doi.org/10.1306/BC74376F-16BE-11D7-8645000102C1865D, 1962.
Baars, D. L. and Stevenson, G. M.: Tectonic evolution of the Paradox Basin, Utah & Colorado, in: Rocky Mountains Association of Geologists, edited by: Wiegand, D. L., Geology of the Paradox Basin, 23–31 pp., ISSN 01675648, 1981.
Baker, A. A.: Geology of the Monument Valley- Navajo Mountain region, San Juan County, Utah, U.S. Geological Survey Bulletin, 865, 1–106, https://doi.org/10.3133/b865, 1936.
Baker, A. A. and Reeside Jr., J. B.: Correlation of the Permian of southern Utah, northern Arizona, northwestern New Mexico, and southwestern Colorado, Am. Assoc. Pet. Geol. Bull., 13, 1413–1448, https://doi.org/10.1306/3D932893-16B1-11D7-8645000102C1865D, 1929.
Beauchamp, B., Henderson, C. M., Dehari, E., Waldbott von Bassenheim, D., Elliot, S., and González, D. C.: Carbonate sedimentology and conodont biostratigraphy of late Pennsylvanian-early Permian stratigraphic sequences, Carlin Canyon Nevada: new insights into the tectonic and oceanographic significance of an iconic succession of the Basin and Range, SEPM Spec. Pub., 113, 34–71, https://doi.org/10.2110/sepmsp.113.14, 2022.
Berner, R. A., VandenBrooks, J. M., and Ward, P. D.: Oxygen and evolution, Science, 316, 557–558, https://doi.org/10.1126/science.1140273, 2007.
Billingsley, G. H. and Huntoon, P. W.: Geologic map of Canyonlands National Park and vicinity, Utah, edited by: Breed, W. J., Canyonlands Natural History Association, 1982.
Blakey, R. C.: Pennsylvanian-Jurassic sedimentary basins of the Colorado Plateau and southern Rocky Mountains, in: The Sedimentary Basins of the United States and Canada, Elsevier, 315–367, https://doi.org/10.1016/B978-0-444-63895-3.00007-3, 2019.
Blanchard, B.: Dept of the Interior Comments on Environmental Assessments for the Davis Canyon Site, Washington D.C., 1–64 pp., https://www.nrc.gov/docs/ML0320/ML032050695.pdf (last access: 11 October 2023), 1985.
Boardman, D. R., Wardlaw, B. R., and Nestell, M. K.: Stratigraphy and conodont biostratigraphy of the uppermost Carboniferous and Lower Permian from the North American Midcontinent, Kansas Geological Survey Bulletin, 255, 1–146, 2009.
Boy, J. A. and Fichter, J.: Stratigraphy of the Saar-Nahe Rotliegendes (? Upper Carboniferous – Lower Permian; Germany), Z. Dtsch. Geol. Ges., 133, 607–642, https://doi.org/10.1127/ZDGG/133/1982/607, 1982.
Brocklehurst, N., Ruta, M., Müller, J., and Fröbisch, J.: Elevated extinction rates as a trigger for diversification rate shifts: early amniotes as a case study, Sci. Rep., 5, 17104, https://doi.org/10.1038/srep17104, 2015.
Carpenter, K. and Ottinger, L.: Permo-Pennsylvanian shark teeth from the lower Cutler beds near Moab, Utah, Geol. Intermount. West, 5, 105–116, https://doi.org/10.31711/giw.v5.pp105-116, 2018.
Chen, J., Montañez, I. P., Qi, Y., Shen, S., and Wang, X.: Strontium and carbon isotopic evidence for decoupling of pCO2 from continental weathering at the apex of the late Paleozoic glaciation, Geology, 46, 395–398, https://doi.org/10.1130/G40093.1, 2018.
Chernykh, V. V. and Ritter, S. M.: Streptognathodus (Conodonta) succession at the proposed Carboniferous-Permian boundary stratotype section, Aidaralash Creek, northern Kazakhstan, J. Paleontol., 71, 459–474, https://doi.org/10.1017/S0022336000039470, 1997.
Chernykh, V. V., Ritter, S. M., and Wardlaw, B. R.: Streptognathodus isolatus new species (Conodonta): proposed index for the Carboniferous-Permian boundary, J. Paleontol., 71, 162–164, https://doi.org/10.1017/S0022336000039068, 1997.
Cleal, C. J. and Thomas, B. A.: Palaeozoic tropical rainforests and their effect on global climates: is the past the key to the present?, Geobiology, 3, 13–31, https://doi.org/10.1111/j.1472-4669.2005.00043.x, 2005.
Cleal, C. L. and Thomas, B. A.: Tectonics, tropical forest destruction and global warming in the late Palaeozoic, Acta Palaeobotanica, Supplement, 39, 17–19, 1999.
Clyde, W. C., Gingerich, P. D., Wing, S. L., Röhl, U., Westerhold, T., Bowen, G., Johnson, K., Baczynski, A. A., Diefendorf, A., McInerney, F., Schnurrenberger, D., Noren, A., Brady, K., and the BBCP Science Team: Bighorn Basin Coring Project (BBCP): a continental perspective on early Paleogene hyperthermals, Sci. Dril., 16, 21–31, https://doi.org/10.5194/sd-16-21-2013, 2013.
Cohen, A., Campisano, C., Arrowsmith, R., Asrat, A., Behrensmeyer, A. K., Deino, A., Feibel, C., Hill, A., Johnson, R., Kingston, J., Lamb, H., Lowenstein, T., Noren, A., Olago, D., Owen, R. B., Potts, R., Reed, K., Renaut, R., Schäbitz, F., Tiercelin, J.-J., Trauth, M. H., Wynn, J., Ivory, S., Brady, K., O'Grady, R., Rodysill, J., Githiri, J., Russell, J., Foerster, V., Dommain, R., Rucina, S., Deocampo, D., Russell, J., Billingsley, A., Beck, C., Dorenbeck, G., Dullo, L., Feary, D., Garello, D., Gromig, R., Johnson, T., Junginger, A., Karanja, M., Kimburi, E., Mbuthia, A., McCartney, T., McNulty, E., Muiruri, V., Nambiro, E., Negash, E. W., Njagi, D., Wilson, J. N., Rabideaux, N., Raub, T., Sier, M. J., Smith, P., Urban, J., Warren, M., Yadeta, M., Yost, C., and Zinaye, B.: The Hominin Sites and Paleolakes Drilling Project: inferring the environmental context of human evolution from eastern African rift lake deposits, Sci. Dril., 21, 1–16, https://doi.org/10.5194/sd-21-1-2016, 2016.
Condon, S. M.: Geology of the Pennsylvanian and Permian Cutler Group and Permian Kaibab Limestone in the Paradox Basin, southeastern Utah and southwestern Colorado, U.S. Geological Survey Bulletin, 2000-P, 1–59, https://doi.org/10.3133/b00P, 1997.
Craddock, K. W., Hook, R. W., Cummins, W. F., Sternberg, C. H., and Olson, E. C.: An overview of vertebrate collecting in the Permian System of north-central Texas, in: Permo-Carboniforous Vertebrate Paleontology, Lithostratigraphy, and Depositional Environments of North-Central Texas, 40–46, Society of Vertebrate Paleontology, 1989.
Cross, W. and Spencer, A. C.: Geology of the Rico Mountains, Colorado, 21st Annual Report of the United States Geological Survey to the Secretary of the Interior, 1899–1900: Part II – General Geology, Economic Geology, Alaska, Government Printing Office, Washington, D.C, 1900.
DiMichele, W. A., Cecil, C. B., Chaney, D. S., Elrick, S. D., and Nelson, W. J.: Fossil floras from the Pennsylvanian-Permian Cutler Group of southeastern Utah, in: Geology of Utah's Far South: Utah Geological Association Publication, Vol. 43, 491–504, 2014.
Doelling, H. H.: Geologic map of the Fisher Towers 7.5' quadrangle, Grand County, Utah, Utah Geological Survey Map, 183, 1–26, https://doi.org/10.34191/m-183, 2002.
Doelling, H. H., Ross, M. L., and Mulvey, W. E.: Geologic map of the Moab 7.5' quadrangle, Grand County, Utah, Utah Geological Survey Map, 181, 1–38, https://doi.org/10.34191/m-181, 2002.
Dubiel, R. F., Huntoon, J. E., Condon, S. M., and Stanesco, J. D.: Permian deposystems, paleogeography, and paleoclimate of the Paradox Basin and vicinity, in: Paleozoic Systems of the Rocky Mountain Region. Society of Economic Paleontologists and Mineralogists, Rocky Mountain Section, edited by: Longman, M. W. and Sonnenfeld, M. D., Denver, 427–443 pp., 1996.
Dubiel, R. F., Huntoon, J. E., Stanesco, J. D., and Condon, S. M.: Cutler Group alluvial, eolian, and marine deposystems: Permian facies relations and climatic variability in the Paradox Basin, Rocky Mountain Association of Geologists, Denver, The Paradox Basin Revisited, 265–308, edited by: Houston, W. S., Wray, L. L., and Moreland, P. G., 2009.
Eberth, D. A., Berman, D. S., Sumida, S. S., and Hopf, H.: Lower Permian terrestrial paleoenvironments and vertebrate paleoecology of the Tambach Basin (Thuringia, central Germany): the upland holy grail, Palaios, 15, 293–313, https://doi.org/10.1669/0883-1351(2000)015<0293:LPTPAV>2.0.CO;2, 2000.
European Commission: Geological Disposal of Radioactive Wastes Produced by Nuclear Power. From Concept to Implementation, Belgium, 43 pp., ISBN 9789289480901, 2004.
Evans, M. and Heller, F.: Environmental Magnetism: Principles and Applications of Enviromagnetics, 1st ed., Elsevier Science, San Diego, CA, 1–299 pp., ISBN 9780080505787, 2003.
Expedition 337 Scientists: in: Methods, edited by: Inagaki, F., Hinrichs, K.-U., Kubo, Y., and the Expedition 337 Scientists, Proc. IODP, 337: Tokyo (Integrated Ocean Drilling Program Management International, Inc.). https://doi.org/10.2204/iodp.proc.337.102.2013, 2013.
Fielding, C. R., Frank, T. D., and Isbell, J. L.: The late Paleozoic ice age – a review of current understanding and synthesis of global climate patterns, Geol. Soc. Am. Spec. Paper, 441, 343–354, https://doi.org/10.1130/2008.2441(24), 2008.
Frederiksen, N. O.: The rise of the mesophytic flora, Geosci. Man, 4, 17–28, https://doi.org/10.2307/3687204, 1972.
Gastaldo, R. A., DiMichele, W. A., and Pfefferkorn, H. W.: Out of the icehouse into the greenhouse: a late Paleozoic analog for modern global vegetational change, GSA Today, 6, 1–7, 1996.
Gay, R. J., Huttenlocker, A. K., Irmis, R. B., Stegner, M. A., and Uglesich, J.: Paleontology of Bears Ears National Monument (Utah, USA): history of exploration, study, and designation, Geol. Intermount. West, 7, 205–241, https://doi.org/10.31711/giw.v7, 2020.
GEO Drilling Fluids, Inc.: About us, https://geodf.com/about-us/ (last access: 22 September 2023), 2023.
Golab, J. A.: The use of ichnofossils in geological and petrophysical characterizations of aquifers and reservoirs: examples from south-central Texas and southeast Utah, Dissertation, University of Kansas, Lawrence, Kansas, 1–195 pp., http://hdl.handle.net/1808/25778 (last access: 10 November 2023), 2016.
Golab, J. A., Smith, J. J., and Hasiotis, S. T.: Paleoenvironmental and paleogeographic implications of paleosols and ichnofossils in the Upper Pennsylvanian Halgaito Formation, southeastern Utah, Palaios, 33, 296–311, https://doi.org/10.2110/palo.2017.074, 2018.
Goldhammer, R. K., Oswald, E. J., and Dunn, P. A.: Hierarchy of stratigraphic forcing: example from Middle Pennsylvanian shelf carbonates of the Paradox Basin, Kansas Geological Survey Bulletin, 233, 361–413, 1991.
Gose, W. A. and Helsley, C. E.: Paleomagnetic and rock-magnetic studies of the Permian Cutler and Elephant Canyon formations in Utah, J. Geophys. Res., 77, 1534–1548, https://doi.org/10.1029/JB077i008p01534, 1972.
Gregory, H. E.: The San Juan country: a geographic and geologic reconnaissance of southeastern Utah, U.S. Geological Survey Professional Paper 188, 1–123, 1938.
Guthrie, J. M. and Bohacs, K. M.: Spatial variability of source rocks: a critical element for defining the petroleum system of Pennsylvanian carbonate reservoirs of the Paradox Basin, SE Utah, RMAG Special Publication, Denver, edited by: Houston, W. S., Wray, L. L., and Moreland, P. G., 2009.
Hansen, F. D. and Leigh, C. D.: Salt Disposal of Heat-Generating Nuclear Waste, Albuquerque, New Mexico, U.S. Department of Energy Office of Scientific and Technical Information Technical Report, 1–110 pp., https://doi.org/10.2172/1005078, 2011.
Henderson, C. M.: Permian conodont biostratigraphy, Geological Society of London Special Publications, 450, 119–142, https://doi.org/10.1144/SP450.9, 2018.
Henderson, C. M., Pinard, S., and Beauchamp, B.: Biostratigraphic and sequence stratigraphic relationships of Upper Carboniferous conodont and foraminifer distribution, Canadian Arctic Archipelago, Bull. Can. Petrol. Geol., 43, 226–246, https://doi.org/10.35767/GSCPGBULL.43.2.226, 1995.
Hintze, L. F., Willis, G. C., Laes, D. Y. M., Sprinkel, D. A., and Brown, K. D.: Digital geologic map of Utah, Utah Geological Survey, 2000.
Hite, R. J. and Buckner, D. H.: Stratigraphic correlations, facies concepts, and cyclicity in Pennsylvanian rocks of the Paradox Basin, in: Rocky Mountain Association of Geologists, Geology of the Paradox Basin, edited by: Wiegand, D. L., 147–159, 1981.
Horton, D. E., Poulsen, C. J., Montañez, I. P., and DiMichele, W. A.: Eccentricity-paced late Paleozoic climate change, Palaeogeogr. Palaeoclimatol. Palaeoecol., 331–332, https://doi.org/10.1016/j.palaeo.2012.03.014, 2012.
Hounslow, M. W. and Balabanov, Y. P.: A geomagnetic polarity timescale for the Permian, calibrated to stage boundaries, Geological Society of London Special Publications, 450, 63–103, https://doi.org/10.1144/SP450.8, 2016.
Huttenlocker, A. K., Henrici, A., Nelson, W. J., Elrick, S., Berman, D. S., Schlotterbeck, T., and Sumida, S. S.: A multitaxic bonebed near the Carboniferous–Permian boundary (Halgaito Formation, Cutler Group) in Valley of the Gods, Utah, USA: vertebrate paleontology and taphonomy, Palaeogeogr. Palaeoclimatol. Palaeoecol., 499, 72–92, https://doi.org/10.1016/j.palaeo.2018.03.017, 2018.
Huttenlocker, A. K., Henderson, C. M., Berman, D. S., Elrick, S. D., Henrici, A. C., and Nelson, W. J.: Carboniferous–Permian conodonts and the age of the lower Cutler Group in the Bears Ears National Monument and vicinity, Utah, USA, Lethaia, 54, 330–340, https://doi.org/10.1111/let.12405, 2021.
Interagency Review Group on Nuclear Waste Management: Report to the President by the Interagency Review Group on Nuclear Waste Management, Washington, DC (USA), 90 pp., 1978.
Isbell, J. L., Henry, L. C., Gulbranson, E. L., Limarino, C. O., Fraiser, M. L., Koch, Z. J., Ciccioli, P. L., and Dineen, A. A.: Glacial paradoxes during the late Paleozoic ice age: evaluating the equilibrium line altitude as a control on glaciation, Gondwana Research, 22, 1–19, https://doi.org/10.1016/j.gr.2011.11.005, 2012.
Ito, E., Higgins, S., Jenkins, C., Leigh, J., Johnson, A., and Grivna, B.: Corelyzer. v2.2.2, Continental Scientific Drilling Facility, Minneapolis, https://github.com/corewall/corelyzer (last access: 15 January 2024), 2023
Jordan, O. D. and Mountney, N. P.: Styles of interaction between aeolian, fluvial and shallow marine environments in the Pennsylvanian to Permian lower Cutler beds, south-east Utah, USA, Sedimentology, 57, 1357–1385, https://doi.org/10.1111/j.1365-3091.2010.01148.x, 2010.
Jordan, O. D. and Mountney, N. P.: Sequence stratigraphic evolution and cyclicity of an ancient coastal desert system: the Pennsylvanian-Permian lower Cutler beds, Paradox Basin, Utah, U.S.A., J. Sediment. Res., 82, 755–780, https://doi.org/10.2110/jsr.2012.54, 2012.
Keeney, R. L.: An analysis of the portfolio of sites to characterize for selecting a nuclear repository, Risk Anal., 7, 195–218, https://doi.org/10.1111/j.1539-6924.1987.tb00982.x, 1987.
Kent, D. V. and Muttoni, G.: Pangea B and the Late Paleozoic Ice Age, Palaeogeogr. Palaeoclimatol. Palaeoecol., 553, 109753, https://doi.org/10.1016/j.palaeo.2020.109753, 2020.
Kent, D. V., Olsen, P. E., and Muttoni, G.: Astrochronostratigraphic polarity time scale (APTS) for the Late Triassic and Early Jurassic from continental sediments and correlation with standard marine stages, Earth Sci. Rev., 166, 153–180, https://doi.org/10.1016/j.earscirev.2016.12.014, 2017.
Kent, D. V., Olsen, P. E., Rasmussen, C., Lepre, C., Mundil, R., Irmis, R. B., Gehrels, G. E., Giesler, D., Geissman, J. W., and Parkerh, W. G.: Empirical evidence for stability of the 405-kiloyear Jupiter-Venus eccentricity cycle over hundreds of millions of years, P. Natl. Acad. Sci. USA, 115, 6153–6158, https://doi.org/10.1073/pnas.1800891115, 2018.
Korte, C. and Ullmann, C. V.: Permian strontium isotope stratigraphy, Geological Society of London Special Publications, 450, 105–118, https://doi.org/10.1144/SP450.5, 2018.
Korte, C., Jasper, T., Kozur, H. W., and Veizer, J.: record of Permian seawater, Palaeogeogr. Palaeoclimatol. Palaeoecol., 240, 89–107, https://doi.org/10.1016/j.palaeo.2006.03.047, 2006.
Langford, R. and Chan, M. A.: Flood surfaces and deflation surfaces within the Cutler Formation and Cedar Mesa Sandstone (Permian), southeastern Utah, Bull. Geol. Soc. Am., 100, 1541–1549, https://doi.org/10.1130/0016-7606(1988)100<1541:FSADSW>2.3.CO;2, 1988.
Langford, R. P. and Salsman, A.: Facies geometries and climatic influence on stratigraphy in the eolian-sabkha transition in the Permian Cedar Mesa Sandstone, SE Utah, Utah Geological Association Publication 43, 275–294, 2014.
Lantink, M. L., Davies, J. H. F. L., Mason, P. R. D., Schaltegger, U., and Hilgen, F. J.: Climate control on banded iron formations linked to orbital eccentricity, Nat. Geosci., 12, 369–374, https://doi.org/10.1038/s41561-019-0332-8, 2019.
Lewis, R. Q., Campbell, R. H., Thaden, R. E., Krummel, W. J. Jr., Willis, G. C., and Matyjasik B.: Geologic map of Elk Ridge and vicinity, San Juan County, Utah (modified from U.S. Geological Survey Professional Paper 474-B), Utah Geological Survey Miscellaneous Publication, 11–1DM, 1–13, https://doi.org/10.34191/mp-11-1dm, 2011.
Liu, Q., Roberts, A. P., Larrasoaa, J. C., Banerjee, S. K., Guyodo, Y., Tauxe, L., and Oldfield, F.: Environmental magnetism: principles and applications, Rev. Geophys., 50, RG4002, https://doi.org/10.1029/2012RG000393, 2012.
Loope, D. B.: Eolian origin of Upper Paleozoic sandstones, southeastern Utah, J. Sediment. Petrol., 54, 563–580, https://doi.org/10.1306/212F846D-2B24-11D7-8648000102C1865D, 1984.
Loope, D. B. and Watkins, D. K.: Pennsylvanian fossils replaced by red chert: early oxidation of pyritic precursors, J. Sediment. Petrol., 59, 375–386, https://doi.org/10.1306/212F8F99-2B24-11D7-8648000102C1865D, 1989.
Loope, D. B., Sanderson, G. A., and Verville, G. J.: Abandonment of the name “Elephant Canyon Formation” in southeastern Utah: physical and temporal implications, Mountain Geologist, 27, 119–130, 1990.
McArthur, J. M., Howarth, R. J., Shields, G. A., and Zhou, Y.: Strontium isotope stratigraphy, in: Geologic Time Scale 2020, edited by: Gradstein, F. M., Ogg, J. G., Schmitz, M. D., and Ogg, G. M., 211–238, Elsevier, Amsterdam, 2020.
McCleary, J.: Characterization of the Davis Canyon site, San Juan County, Utah, as a potential repository for the disposal of high level nuclear waste and spent fuel, Utah Geologic Association: Geology and Hydrology of Hazardous-Waste, Mining-Waste, Water-Waste, and Repository Sites in Utah, 209–222, https://doi.org/10.1016/0148-9062(91)91093-7, 1989.
Merkhofer, M. W. and Keeney, R. L.: A multiattribute utility analysis of alternative sites for the disposal of nuclear waste, Risk Anal., 7, 173–194, https://doi.org/10.1111/j.1539-6924.1987.tb00981.x, 1987.
Michel, L. A., Tabor, N. J., Montañez, I. P., Schmitz, M. D., and Davydov, V. I.: Chronostratigraphy and paleoclimatology of the Lodève Basin, France: evidence for a pan-tropical aridification event across the Carboniferous–Permian boundary, Palaeogeogr. Palaeoclimatol. Palaeoecol., 430, 118–131, https://doi.org/10.1016/J.PALAEO.2015.03.020, 2015.
Montañez, I. and Soreghan, G. S.: Earth's fickle climate: lessons learned from deep-time ice ages, Geotimes, 51, 24–27, http://www.geotimes.org/mar06/feature_deeptimeiceages.html (last access: 10 November 2023), 2006.
Montañez, I. P.: A Late Paleozoic climate window of opportunity, P. Natl. Acad. Sci. USA, 113, 2334–2336, https://doi.org/10.1073/pnas.1600236113, 2016.
Montañez, I. P. and Poulsen, C. J.: The late Paleozoic ice age: an evolving paradigm, Annu. Rev. Earth Planet Sci., 41, 629–656, https://doi.org/10.1146/annurev.earth.031208.100118, 2013.
Moore, K. D., Soreghan, G. S., and Sweet, D. E.: Stratigraphic and structural relations in the proximal Cutler Formation of the Paradox Basin: implications for timing of movement on the Uncompahgre Front, Mountain Geologist, 45, 49–68, 2008.
Mountney, N. P.: Periodic accumulation and destruction of aeolian erg sequences in the Permian Cedar Mesa Sandstone, White Canyon, southern Utah, USA, Sedimentology 53, 789–823, 2006.
Mountney, N. P. and Jagger, A.: Stratigraphic evolution of an aeolian erg margin system: the Permian Cedar Mesa Sandstone, SE Utah, USA, Sedimentology, 51, 713–743, https://doi.org/10.1111/j.1365-3091.2004.00646.x, 2004.
Murphy, K.: Eolian origin of upper Paleozoic red siltstones at Mexican Hat and Dark Canyon, southeastern Utah, Master's Thesis, University of Nebraska-Lincoln, Lincoln, Nebraska, 1–138 pp., 1987.
Nail, R. S.: Middle-Late Pennsylvanian fusulinid faunas from midcontinent North America and the Paradox Basin, Utah and Colorado, Dissertation, Texas Tech University, Lubbock, Texas, 1–371 pp., ISBN 9798641029290, 1996.
Nail, R. S., Barrick, J. E., and Williams, M. R.: Fusulinid and conodont biostratigraphy of sedimentary cycles in the middle and upper Pennsylvanian Honaker Trail Formation, western Paradox Basin, GSA Abstracts with Programs, 26, 56, 1994
Nail, R. S., Barrick, J. E., and Ritter, S. M.: Preliminary fusulinid and condodont biostratigraphy of the Honaker Trail Formation (late Middle Pennslvanian-Late Pennsylvanian) in the Gibson Dome 1 and Elk Ridge 1 cores, Paradox Basin, Utah Geological Association Guidebook, 25, 303–312, https://archives.datapages.com/data/uga/data/067/067001/303_ugs670303.htm (last access: 10 November 2023), 1996.
Olivier, M., Bourquin, S., Desaubliaux, G., Ducassou, C., Rossignol, C., Daniau, G., and Chaney, D.: The Late Paleozoic Ice Age in western equatorial Pangea: context for complex interactions among aeolian, alluvial, and shoreface sedimentary environments during the Late Pennsylvanian – early Permian, Gondwana Res., 124, 305–338, 2023.
Olsen, P. E. and Kent, D. V.: Long-period Milankovitch cycles from the Late Triassic and Early Jurassic of eastern North America and their implications for the calibration of the Early Mesozoic time–scale and the long–term behaviour of the planets, Philos. Trans. Roy. Soc. London A, 357, 1761–1786, https://doi.org/10.1098/rsta.1999.0400, 1999.
Olsen, P. E., Geissman, J. W., Kent, D. V., Gehrels, G. E., Mundil, R., Irmis, R. B., Lepre, C., Rasmussen, C., Giesler, D., Parker, W. G., Zakharova, N., Kürschner, W. M., Miller, C., Baranyi, V., Schaller, M. F., Whiteside, J. H., Schnurrenberger, D., Noren, A., Brady Shannon, K., O'Grady, R., Colbert, M. W., Maisano, J., Edey, D., Kinney, S. T., Molina-Garza, R., Bachman, G. H., Sha, J., and the CPCP team: Colorado Plateau Coring Project, Phase I (CPCP-I): a continuously cored, globally exportable chronology of Triassic continental environmental change from western North America, Sci. Dril., 24, 15–40, https://doi.org/10.5194/sd-24-15-2018, 2018.
Olsen, P. E., Laskar, J., Kent, D. V., Kinney, S. T., Reynolds, D. J., Sha, J., and Whiteside, J. H.: Mapping Solar System chaos with the geological orrery, P. Natl. Acad. Sci. USA, 116, 10664–10673, 2019.
Olson, E. C. and Vaughn, P. P.: The changes of terrestrial vertebrates and climates during the Permian of North America, Forma et functio, 3, 113–138, 1970.
Orkild, P. P.: Photogeologic map of the Bluff-6 quadrangle, San Juan County, Utah, U.S. Geological Survey IMAP, 53, https://doi.org/10.3133/i53, 1955.
O'Sullivan, R. B.: Geology of the Cedar Mesa-Boundary Butte area, San Juan County, Utah, U.S. Geological Survey Bulletin, 1186, 1–128, https://doi.org/10.3133/b1186, 1965.
Pardo, J. D., Small, B. J., Milner, A. R., and Huttenlocker, A. K.: Carboniferous–Permian climate change constrained early land vertebrate radiations, Nat. Ecol. Evol., 3, 200–206, https://doi.org/10.1038/s41559-018-0776-z, 2019.
Parrish, W. C.: Paleoenvironmental analysis of a Lower Permian bonebed and adjacent sediments, Wichita County, Texas, Palaeogeogr. Palaeoclimatol. Palaeoecol., 24, 209–237, https://doi.org/10.1016/0031-0182(78)90043-3, 1978.
Pearson, M. R., Benson, R. B. J., Upchurch, P., Fröbisch, J., and Kammerer, C. F.: Reconstructing the diversity of early terrestrial herbivorous tetrapods, Palaeogeogr. Palaeoclimatol. Palaeoecol., 372, 42–49, https://doi.org/10.1016/j.palaeo.2012.11.008, 2013.
Peterson, J. A. and Hite, R. J.: Pennsylvanian evaporite-carbonate cycles and their relation to petroleum occurrence, southern Rocky Mountains, Am. Assoc. Pet. Geol. Bull., 53, 884–908, https://doi.org/10.1306/5d25c807-16c1-11d7-8645000102c1865d, 1969.
Petrychenko, O. Y., Williams-Stroud, S. C., and Peryt, T. M.: The relationship of brine chemistry of the Pennsylvanian Paradox evaporite basin (southwestern USA) to secular variation in seawater chemistry, Geological Quarterly, 56, 25–40, 2012.
Pettigrew, R. P., Priddy, C., Clarke, S. M., Warke, M. R., Stüeken, E. E., and Claire, M. W.: Sedimentology and isotope geochemistry of transitional evaporitic environments within arid continental settings: from erg to saline lakes, Sedimentology, 68, 907–942, https://doi.org/10.1111/sed.12816, 2021.
Pfeifer, L. S., Hinnov, L., Zeeden, C., Rolf, C., Laag, C., and Soreghan, G. S.: Rock magnetic cyclostratigraphy of Permian loess in eastern equatorial Pangea (Salagou Formation, south-central France), Front. Earth Sci., 8, 241, 1–13, https://doi.org/10.3389/feart.2020.00241, 2020.
Pierce, W. G. and Rich, E. I.: Summary of rock salt deposits in the United States as possible storage sites for radioactive waste materials, US Geological Survey Bulletin 1148, 1–91, 1962.
Rasmussen, D. L. and Rasmussen, L.: Regional cross sections of the Paradox Basin, in: Subsurface Cross Sections of Southern Rocky Mountains, edited by: Rasmussen, L., Payne, J., and Curnella, S. P., Rocky Mountain Association of Geologists, Denver, 24–27, 2018.
Raup, O. B. and Hite, R. J.: Bromine geochemistry of chloride rocks of the Middle Pennsylvanian Paradox Formation of the Hermosa Group, Paradox Basin, Utah and Colorado, US Geological Survey Bulletin, 2000 M, 1–117, https://doi.org/10.3133/b00M, 1996.
Reed, J. A., Cervato, C., and Fils, D.: PSICAT: a new open-source core description application, in: The Paleontological Stratigraphic Interval Construction and Analysis Tool, Iowa State University, Ames, Iowa, 15–22, 2007.
Reese, A.: The bones of Bears Ears, Science, 363, 218–220, https://doi.org/10.1126/science.363.6424.218, 2019.
Reisz, R. R. and Fröbisch, J.: The oldest caseid synapsid from the late Pennsylvanian of Kansas, and the evolution of herbivory in terrestrial vertebrates, PLoS One, 9, e94518, https://doi.org/10.1371/journal.pone.0094518, 2014.
Reisz, R. R. and Sues, H.-D.: Herbivory in late Paleozoic and Triassic terrestrial vertebrates, in: Evolution of Herbivory in Terrestrial Vertebrates, Cambridge University Press, edited by: Sues, H.-D., 9–41, https://doi.org/10.1017/cbo9780511549717.003, 2000.
Ritter, S. M.: Upper Missourian–lower Wolfcampian (upper Kasimovian–lower Asselian) conodont biostratigraphy of the midcontinent, U.S.A., J. Paleontol., 69, 1139–1154, https://doi.org/https://doi.org/10.1017/S0022336000038129, 1995.
Ritter, S. M., Barrick, J. E., and Skinner, M. R.: Conodont sequence biostratigraphy of the Hermosa Group (Pennsylvanian) at Honaker Trail, Paradox Basin, Utah, J. Paleontol., 76, 495–517, https://doi.org/10.1017/S0022336000037331, 2002.
Romer, A. S.: The late Carboniferous vertebrate fauna of Kounova (Bohemia) compared with that of the Texas redbeds, Am. J. Sci., 243, 417–442, https://doi.org/10.2475/AJS.243.8.417, 1945.
Rowley, D. B., Raymond, A., Parrish, J. T., Lottes, A. L., Scotese, C. R., and Ziegler, A. M.: Carboniferous paleogeographic, phytogeographic, and paleoclimatic reconstructions, Int. J. Coal. Geol., 5, 7–42, https://doi.org/10.1016/0166-5162(85)90009-6, 1985.
Sahney, S., Benton, M. J., and Falcon-Lang, H. J.: Rainforest collapse triggered Carboniferous tetrapod diversification in Euramerica, Geology, 38, 1079–1082, https://doi.org/10.1130/G31182.1, 2010.
Scott, G. R.: Paleomagnetism of Carboniferous and Triassic strata from cratonic North America, Dissertation, The University of Texas at Dallas, Dallas, 1–172 pp., ISBN 9781083468468, 1975.
Scott, K. M.: Carboniferous–Permian boundary in the Halgaito Formation, Cutler Group, Valley of the Gods and surrounding area, southeastern Utah, in: The Carboniferous–Permian Transition, New Mexico Museum of Natural History and Science Bulletin, 60, 398–409, 2013.
Sears, J. D.: Geology of Comb Ridge and vicinity north of San Juan River, San Juan County, Utah, U.S. Geological Survey Bulletin, 1021-D, 167–207, https://doi.org/10.3133/b1021E, 1956.
Soreghan, M. J. and Soreghan, G. S. (Lynn): Whole-rock geochemistry of upper Paleozoic loessite, western Pangaea: implications for paleo-atmospheric circulation, Earth Planet Sci. Lett., 255, 117–132, https://doi.org/10.1016/j.epsl.2006.12.010, 2007.
Soreghan, G. S., Elmore, R. D., and Lewchuk, M. T.: Sedimentologic-magnetic record of western Pangean climate in upper Paleozoic loessite (lower Cutler beds, Utah), Geol. Soc. Am. Bull., 114, 1019–1035, 2002a.
Soreghan, M. J., Soreghan, G. S. (Lynn), and Hamilton, M. A.: Paleowinds inferred from detrital-zircon geochronology of upper Paleozoic loessite, western equatorial Pangea, Geology, 30, 695–698, https://doi.org/10.1130/0091-7613(2002)030<0695:PIFDZG>2.0.CO;2, 2002b.
Soreghan, G. S., Soreghan, M. J., and Hamilton, M. A.: Origin and significance of loess in late Paleozoic western Pangaea: a record of tropical cold?, Palaeogeogr. Palaeoclimatol. Palaeoecol., 268, 234–259, https://doi.org/10.1016/j.palaeo.2008.03.050, 2008.
Soreghan, G. S., Beccaletto, L., Benison, K. C., Bourquin, S., Feulner, G., Hamamura, N., Hamilton, M., Heavens, N. G., Hinnov, L., Huttenlocker, A., Looy, C., Pfeifer, L. S., Pochat, S., Sardar Abadi, M., Zambito, J., and the Deep Dust workshop participants: Report on ICDP Deep Dust workshops: probing continental climate of the late Paleozoic icehouse–greenhouse transition and beyond, Sci. Dril., 28, 93–112, https://doi.org/10.5194/sd-28-93-2020, 2020.
Soreghan, G. S., Heavens, N. G., Pfeifer, L. S., and Soreghan, M. J.: Dust and loess as archives and agents of climate and climate change in the late Paleozoic Earth system, Geological Society, London, Special Publications, 535, 195–223, https://doi.org/10.1144/sp535-2022-208, 2023.
Stanesco, J. D. and Campbell, J. A.: Eolian and noneolian facies of the Lower Permian Cedar Mesa Sandstone Member of the Cutler Formation, southeastern Utah, U.S. Geological Survey Bulletin, 1808-F, 1–13 https://doi.org/10.3133/b1808EF, 1989.
Stuckless, J. S. and Levich, R. A.: The road to Yucca Mountain – evolution of nuclear waste disposal in the United States, Environ. Eng. Geosci., 22, 1–25, https://doi.org/10.2113/gseegeosci.22.1.1, 2016.
Sues, H. D. and Reisz, R. R.: Origins and early evolution of herbivory in tetrapods, Trends in Ecology & Evolution, 13, 141–145, https://doi.org/10.1016/S0169-5347(97)01257-3, 1998.
Sweet, D. E., Brotherton, J. L., Chowdhury, N. U. M. K., and Ramsey, C. E.: Tectonic subsidence analysis of the Ancestral Rocky Mountains from the interior to the southern margin, Palaeogeogr. Palaeoclimatol. Palaeoecol., 576, 110508, https://doi.org/10.1016/j.palaeo.2021.110508, 2021.
Tabor, N. J. and Poulsen, C. J.: Palaeoclimate across the Late Pennsylvanian-Early Permian tropical palaeolatitudes: a review of climate indicators, their distribution, and relation to palaeophysiographic climate factors, Palaeogeogr. Palaeoclimatol. Palaeoecol., 268, 293–310, https://doi.org/10.1016/j.palaeo.2008.03.052, 2008.
Thackston, J. W., Preslo, L. M., Hoexter, D. E., and Donnelly, N.: Results of hydraulic tests at Gibson Dome No. 1, Elk Ridge No. 1, and E. J. Kubat boreholes, Paradox Basin, Utah, Office of Nuclear Waste Isolation Technical Report, Battelle Memorial Institute, Columbus, 1–99, 1984.
Tobey, D. E.: Water and Wind: The fluvial and eolian forces behind the Pennsylvanian-Permian Halgaito Formation, Utah, Dissertation, Dalhousie University, Halifax, Nova Scotia, 1–103 pp., http://hdl.handle.net/10222/79933 (last access: 10 November 2023), 2020.
Tromp, D. E.: Clays as indicators of depositional and diagenetic conditions in Pennsylvanian black shales, Paradox Basin, Utah and Colorado, Dissertation, Colorado School of Mines, Golden, Colorado, 1–168 pp., https://hdl.handle.net/11124/176268 (last access: 10 November 2023), 1995.
Tuttle, M. L., Klett, T. R., Richardson, M., and Breit, G. N.: Geochemistry of two interbeds in the Pennsylvanian Paradox Formation, Utah and Colorado – a record of deposition and diagenesis of repetitive cycles in a marine basin, US Geological Survey Bulletin, 2000-N, 1–86, https://doi.org/10.3133/b00N, 1996.
Uhl, D., Lausberg, S., Noll, R., and Stapf, K. R. G.: Wildfires in the late Palaeozoic of central Europe – an overview of the Rotliegend (Upper Carboniferous–Lower Permian) of the Saar–Nahe Basin (SW-Germany), Palaeogeogr. Palaeoclimatol. Palaeoecol., 207, 23–35, https://doi.org/10.1016/J.PALAEO.2004.01.019, 2004.
U.S. Department of Energy: Environmental Assessment Overview: Davis Canyon Site, Utah, Washington D.C., 38 pp., 1986.
van Hinsbergen, D. J. J., de Groot, L. V, van Schaik, S. J., Spakman, W., Bijl, P. K., Sluijs, A., Langereis, C. G., and Brinkhuis, H.: A paleolatitude calculator for paleoclimate studies, PLoS One, 10, e0126946, https://doi.org/10.1371/journal.pone.0126946, 2015.
Vaughn, P. P.: Comparison of the Early Permian vertebrate faunas of the Four Corners region and north-central Texas, Los Angeles County Museum Contributions in Science, 105, 1–13, https://doi.org/10.5962/p.241094, 1966.
Vaughn, P. P.: Lower Permian vertebrates of the Four Corners and the Midcontinent as indices of climatic differences, in: Proceedings of the North American Paleontological Congress, edited by: Yochelson, E. L., Vol. 1969, Allen Press, Lawrence, KS, USA, 388–408, 1970.
Veizer, J.: Strontium isotopes in seawater through time, Annu. Rev. Earth Planet Sci., 17, 141–167, https://doi.org/10.1146/annurev.ea.17.050189.001041, 1989.
Verosub, K. L. and Roberts, A. P.: Environmental magnetism: past, present, and future, J. Geophys. Res., 100, 2175–2192, https://doi.org/10.1029/94JB02713, 1995.
Wardlaw, B. R.: Age assignment of the Pennsylvanian-Early Permian succession of north central Texas, Permophiles, 46, 21–22, 2005.
Wardlaw, B. R. and Nestell, M. K.: The first appearance of Streptognathodus isolatus in the Permian of Texas, Permophiles, 59, 17–20, 2014.
Wengerd, S. A.: Pennsylvanian Stratigraphy, Southwest Shelf, Paradox Basin, Intermountain Association of Petroleum Geologists: Guidebook to the Geology of the Paradox Basin, 109–134, 1958.
Wengerd, S. A.: Stratigraphic section at Honaker Trail, San Juan Canyon, San Juan County, Utah, Four Corners Geological Society Guidebook, 4, 236–243, 1963.
Whidden, K. J., Lillis, P. G., Anna, L. O., Pearson, K. M., and Dubiel, R. F.: Geology and total petroleum systems of the Paradox Basin, Utah, Colorado, New Mexico, and Arizona, The Mountain Geologist, 51, 119–138, 2014.
Williams, M. R.: Stratigraphy of Upper Pennsylvanian cyclic carbonate and siliciclastic rocks, western Paradox Basin, Utah, in: The Paradox Basin Revisited – New Developments in Petroleum Systems and Basin Analysis, edited by: Houston, W. S., Wray, L. L., and Moreland, P. G., Rocky Mountain Association of Geologists, Denver, 381–435, 2009.
Woodward-Clyde Consultants: Completion report for Elk Ridge no. 1 borehole, Elk Ridge study area of the Paradox Basin region, San Juan County, Utah: Topical Report, San Francisco, California, 1–803 pp., 1982a.
Woodward-Clyde Consultants: Geologic Characterization Report for the Paradox Basin Study Region Utah Study Areas – Volume III: Elk Ridge, Woodward-Clyde Consultants, 1–172 pp., 1982b.