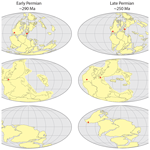
Report on ICDP Deep Dust workshops: probing continental climate of the late Paleozoic icehouse–greenhouse transition and beyond
Laurent Beccaletto
Kathleen C. Benison
Sylvie Bourquin
Georg Feulner
Natsuko Hamamura
Michael Hamilton
Nicholas G. Heavens
Linda Hinnov
Adam Huttenlocker
Cindy Looy
Lily S. Pfeifer
Stephane Pochat
Mehrdad Sardar Abadi
James Zambito
Chamberlin and Salisbury's assessment of the Permian a century ago captured the essence of the period: it is an interval of extremes yet one sufficiently recent to have affected a biosphere with near-modern complexity. The events of the Permian – the orogenic episodes, massive biospheric turnovers, both icehouse and greenhouse antitheses, and Mars-analog lithofacies – boggle the imagination and present us with great opportunities to explore Earth system behavior. The ICDP-funded workshops dubbed “Deep Dust,” held in Oklahoma (USA) in March 2019 (67 participants from nine countries) and Paris (France) in January 2020 (33 participants from eight countries), focused on clarifying the scientific drivers and key sites for coring continuous sections of Permian continental (loess, lacustrine, and associated) strata that preserve high-resolution records. Combined, the two workshops hosted a total of 91 participants representing 14 countries, with broad expertise. Discussions at Deep Dust 1.0 (USA) focused on the primary research questions of paleoclimate, paleoenvironments, and paleoecology of icehouse collapse and the run-up to the Great Dying and both the modern and Permian deep microbial biosphere. Auxiliary science topics included tectonics, induced seismicity, geothermal energy, and planetary science. Deep Dust 1.0 also addressed site selection as well as scientific approaches, logistical challenges, and broader impacts and included a mid-workshop field trip to view the Permian of Oklahoma. Deep Dust 2.0 focused specifically on honing the European target. The Anadarko Basin (Oklahoma) and Paris Basin (France) represent the most promising initial targets to capture complete or near-complete stratigraphic coverage through continental successions that serve as reference points for western and eastern equatorial Pangaea.
- Article
(14971 KB) - Full-text XML
-
Supplement
(75 KB) - BibTeX
- EndNote
Between a marvelous deployment of glaciation, a strangely dispersed deposition of salt and gypsum, an extraordinary development of red beds, a decided change in terrestrial vegetation, a great depletion of marine life, a remarkable shifting of geographic outlines, and a pronounced stage of crustal folding, the events of the Permian period constitute a climacteric combination. Each of these phenomena brings its own unsolved questions, while their combination presents a plexus of problems of unparalleled difficulty. More than any other period since the Proterozoic, the Permian is the period of problems.
Chamberlin and Salisbury, Geology v. II, Earth History, 1905
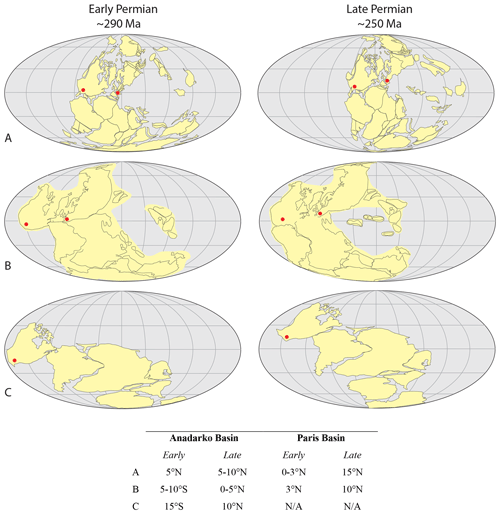
Figure 1Paleogeography during the early to late Permian redrafted from the reconstructions of (a) Domeier and Torsvik (2014), (b) Muttoni and Kent (2019), and (c) Tomezzoli et al. (2018). Red dots show drill target paleo-positions within the equatorial Central Pangaean Mountains (western US – western equatorial Pangaea and western Europe – eastern equatorial Pangaea). A significant difference between (a) and (b) relates to the contrasting reconstructions of Pangaea A and Pangaea B. Pangaea A prevails for the later Permian, whereas some prefer Pangaea B for the early Permian. The reconstruction of Tomezzoli et al. (2018) focuses on Laurentia and Gondwana. In all cases, the target sites are generally equatorial but vary by up to (coordinates for each configuration summarized in the table below).
The Permian (299–252 Ma) records a fundamental reorganization in tectonic, climatic, and biologic components of the Earth system. Plate collisions leading to the assembly of the supercontinent Pangaea culminated by middle Permian time, expressed at low latitudes by the formation of the Central Pangaean Mountains and associated orogenic belts (e.g., Domeier and Torsvik, 2014; Fig. 1). The Permian records multiple large mafic magmatic events, including those affecting the Central Pangaean Mountains (Ernst and Buchan, 2001). Additionally, the Variscan system of western and central Europe was a global focus of expansive and explosive silicic-intermediate volcanism (Soreghan et al., 2019) associated with both construction and collapse of the Central Pangaean Mountains (e.g., Menard and Molnar, 1988; Burg et al., 1994). Extensive plutonism and orogenic collapse also took place in the Moroccan and Appalachian parts of this system (e.g., Valentino and Gates, 2001; EL Hadi et al., 2006). The magnitude of the Permian climate transition is unique for the Phanerozoic: Earth's penultimate global icehouse peaked during the early Permian, yielding to full greenhouse conditions by the late Permian – our only example of icehouse collapse during a time with known complex terrestrial ecosystems (Gastaldo et al., 1996). The late Paleozoic icehouse was the longest and most intense glaciation of the Phanerozoic (Feulner, 2017), and reconstructed atmospheric composition includes both the lowest CO2 and (inferred) highest O2 levels of the Phanerozoic (Berner, 2006; Foster et al., 2017) – the latter presumably spurred by the massive proliferation of vascular land plants (Berner, 2003), possibly exacerbated by weathering of the tropical mountains (Goddéris, 2017) as well as enhanced marine productivity (Sur et al., 2015; Chen et al., 2018; Sardar Abadi et al., 2020). High-resolution CO2 reconstructions include values comparable to those anticipated for Earth's immediate future (Montañez et al., 2016). Fundamental shifts occurred in atmospheric circulation, notably development of a global megamonsoon (Parrish, 1993), and the tropics are inferred to have been anomalously arid (e.g., Tabor and Poulsen, 2008), although the latter could alternatively reflect paleogeographic details that remain poorly resolved (Fig. 1; Domeier and Torsvik, 2014; Tomezzoli et al., 2018; Muttoni and Kent, 2019). Evolution of the terrestrial biosphere during the Carboniferous produced the first tropical rainforests (Cleal and Thomas, 2005) and terrestrial ecosystems of “modern” complexity and structure, with food webs centered on carnivores consuming novel vertebrate herbivores (Sues and Reisz, 1998). By Permian time, extreme environments are well documented in the form of, e.g., voluminous dust deposits (Soreghan et al., 2008b, 2015a), acid saline lakes and groundwaters (Benison et al., 1998), extreme continental temperatures (Zambito and Benison, 2013), and biotic crises and extirpations of spore plants from equatorial Euramerica (DiMichele et al., 2006; Sahney et al., 2010), ultimately culminating at the end of the Permian with the largest extinction event in the Phanerozoic (e.g., Twichett et al., 2001; Erwin, 2006). Figure 2 illustrates major trends in varied aspects of the Permian Earth system.
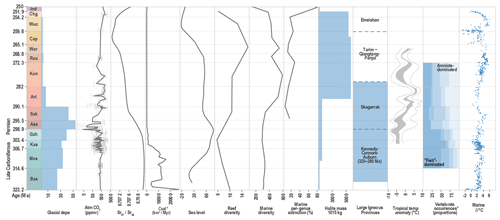
Figure 2Illustration of documented glacial deposits (Soreghan et al., 2019), atmospheric pCO2 (Montañez et al., 2016; Foster et al., 2017), strontium isotope signature (McArthur et al., 2001), terrestrial and North American terrestrial organic sediment (coal, lignite, anthracite, and tar) accumulation* (Nelsen et al., 2016), sea-level changes (Haq and Schutter, 2008), mean species richness of reef builders in 10 Myr bins (Kiessling, 2005; Alroy et al., 2008; Payne and Clapham, 2012), halite mass (Hay et al., 2006; Warren, 2016), and large igneous provinces (LIP Commission) during the late Carboniferous (Pennsylvanian) and Permian (323–251 Ma), proportion of vertebrate (fish versus amniote-dominant) species in the North American continental realm (Pardo et al., 2019), a tropical temperature curve adapted from Montañez et al. (2007), and marine C13 adapted from Saltzmann and Thomas (2012). * indicates North America only, otherwise global signatures.
Table 1European target decision matrix.
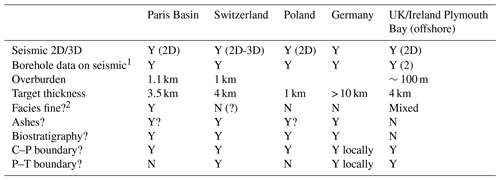
1 Borehole data – within 5 km of proposed location
2 Predominantly (> 80 %) fine facies
The dynamic Earth system of the Permian and the sensitivity of the tropics to climate forcings in particular motivate an international drilling program to focus on recovery of high-resolution continental records from both western and eastern low-latitude Pangaea in addition to auxiliary science objectives. To address this, 67 scientists and students from nine countries gathered in Norman, Oklahoma (USA), on 7–10 March 2019 to discuss plans for an international drilling project to target objectives in both the US and western Europe. The project was dubbed “Deep Dust” for the intended focus on eolian-transported fines, which are interpreted to compose much of the sediment ultimately captured in eolian, lacustrine, and associated depositional environments of the Anadarko Basin of Oklahoma and selected Permian basins of western Europe. The workshop began with overviews of the key science objectives, which included themes on paleoclimate, paleobiology, the microbial biosphere (both modern deep and fossil Earth surface), and auxiliary science, as well as presentations on the regional geology of the Anadarko and western European basins (Table 1). The rest of the workshop focused on breakout groups and summary plenary sessions on topics of scientific methodologies, drilling logistics (site selection, hazards, logging, operations), education and outreach, and funding. Following discussions of science objectives and geology of potential sites, participants took a field excursion to view target Permian strata of the Anadarko Basin, which consist predominantly of very fine-grained red beds and evaporites, with discussions focused on interpretations of the predominance of eolian and lacustrine environments (Giles et al., 2013; Sweet et al., 2013; Foster et al., 2014; M. Soreghan et al., 2018). The field excursion concluded with an unscheduled but quintessentially Oklahoman bovine rescue. A follow-on workshop (dubbed “Deep Dust 2.0”) held in Paris (27–28 January 2020) involved 33 scientists from eight countries in western–central Europe and focused on honing the European coring site(s). After an overview of the regional geology, paleoenvironments, and expected facies in basins of western and central Europe (Polish Basin, Italian basins, German basins, Swiss Plateau, UK and Irish basins, and the French Lodève and Paris basins), presentations (Table 1) focused on topics such as the utility of plant fossils, Permian geothermal resources, and the positions and perspectives of the BRGM (Bureau de Recherches Géologiques et Miniéres) and CNRS (Centre National de la Recherche Scientifique) regarding a proposed research coring project.
The Deep Dust 1.0 workshop (in Oklahoma) included plenary presentations (Table 1) that provided overviews of geologic evidence and modeling results on Permian paleoenvironment and paleoclimates, extreme environments of the Permian, the Permian carbon cycle, terrestrial biosphere and run-up to the Great Dying, and aspects of the deep microbial biosphere. In discussions, participants identified the following major science objectives and rationales for coring these rocks, which are neither well preserved nor very accessible in outcrops, and for which minimal core exists. A list of questions associated with the major and supplementary science topics appears in a data supplement table; overviews appear below. Coring is essential to (1) access buried upland records, (2) achieve continuous records in basinal regions, and (3) recover a pristine section suitable for application of various sedimentologic, geochemical, fluid-inclusion, rock-magnetic, magnetostratigraphic, paleontologic, and geochronologic approaches (i.e., Soreghan et al., 2015b; Benison et al., 2015). Additionally, during the course of both workshops, participants suggested considering expansion to a staged project that could incorporate coring in (1) multiple basins of western Europe and (2) in mid-paleolatitude sites (e.g., China). Such an expanded approach would better inform paleoclimate modeling.
2.1 Equatorial paleoclimate of peak icehouse and icehouse collapse
The basal Permian records the most extreme phase of the late Paleozoic ice age (LPIA), which was the longest-lived and most intense icehouse of the Phanerozoic. Continental glaciation extended to latitudes as low as 32∘ S (Evans, 2003), and the Carboniferous–Permian boundary interval archives the most widespread evidence for glacial deposits of the LPIA (Fielding et al., 2008; Soreghan et al., 2019; Fig. 2). The acme conditions of the Asselian yielded to a massive reduction in ice (as recorded by number of ice-contact deposits) in the Sakmarian, followed by ultimate disappearance of the vestigial Gondwanan glacial deposits (Australia) in the Wuchiapingian age (Fig. 2). The role of pCO2, which reached remarkably low values during the LPIA, is an established factor in the severity of the icehouse, but contributory factors might include negative radiative forcing from volcanic aerosols (Soreghan et al., 2019). Fine-grained loessitic and lacustrine strata of the target regions preserve high-resolution records of paleoclimate in these “far-field” equatorial regions, including lower Permian cyclothemic strata that record unequivocal glacial–interglacial swings, yielding upward to entirely continental successions, such as those seen in the upper Pennsylvanian through lower Permian of mid-continental North America (e.g., Heckel, 1990; Giles et al., 2013). The cause(s) of the LPIA deglaciation remain(s) in debate, in addition to the rate of deglaciation; carbon cycling played a key role, but the mechanisms for changing pCO2 as well as the role(s) of other factors remain(s) debated. Addressing these and related issues will require data types such as additional constraints on high-resolution pCO2, atmospheric dust loading, and records of source-region denudation (from thermochronology). Combining this with a high-resolution Sr isotope curve can help address the relative roles of weathering and other forcing in driving deglaciation and, in the longer term, the apparent aridification of equatorial Pangaea. Our understanding of the aridification hinges on accuracy of paleogeographic reconstructions; although reconstructions vary (Fig. 1), the variation falls largely within of the Equator, suggesting that aridification relates primarily to changing equatorial hydroclimate rather than latitudinal drivers. However, the Pangaea A versus Pangaea B configurations (Domeier and Torsvik, 2014; Tomezzoli et al., 2018; Muttoni and Kent, 2019) for early Permian time have profoundly different implications for the continentality of western Europe, in particular. In principle, such pronounced differences between continental reconstructions could be tested by running climate-model simulations for different reconstructions and comparing simulated precipitation and evaporation patterns with the distribution of lithological indicators such as coal or evaporite (Cao et al., 2019).
The possibility that glaciation affected low–moderate-elevation uplands of both the western (US) and eastern (Europe) equatorial regions (Becq-Giraudon et al., 1996; Soreghan et al., 2008a, 2014; Pfeifer et al., 2020a) in the early Permian remains highly controversial but is potentially testable by coring regions proximal to buried paleo-uplands to seek evidence for ice-contact and/or pro- and peri-glacial deposition. Furthermore, the unique geological setting of the Anadarko Basin records epeirogenic subsidence that preserved buried paleo-highs (Soreghan et al., 2012), thus enabling (via coring) access to upland environments. Low-latitude upland glaciation – if substantiated – could reflect increased glacial-phase albedo from high loading of bright aerosols, greater exposure of bare ground due to vegetation changes during glacials (Falcon-Lang and DiMichele, 2010), or other as-yet undiscovered climate forcings. Evidence for low-latitude glaciation across equatorial Pangaea during the late Paleozoic would radically change our understanding of this period in Earth's history – a critical interval for the evolution of land animals and plants. It would spur research on influences of alternative boundary conditions and climate forcings. Furthermore, changes in erosional conditions imposed by climate can affect surface expression of tectonic processes (e.g., Molnar and England, 1990; Sternai et al., 2012). These inter-relations are even more intriguing given glaciation, as ice growth and associated erosion affect uplift and subsidence (e.g., Molnar and England, 1990; Champagnac et al., 2009).
2.2 Atmospheric dust and the Pangaean megamonsoon
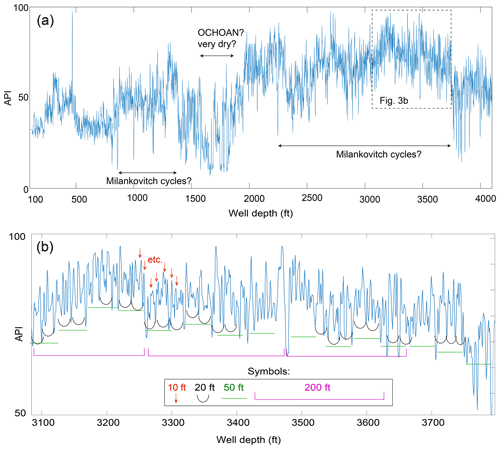
Figure 3Gamma ray (GR) log from the Schneberger 1-19 Well, Atoka Burns Flat, Washita County, Oklahoma, USA (S19; T10N, R19W; elevation 593 m). (a) Log from 100 to 4100 ft (well log depth scale) with preliminary interpretations of intervals with possible Milankovitch cyclicity and possible position of the North American Ochoan Stage (earliest Lopingian). The dashed box indicates a detailed portion of the log examined further in (b). (b) Detail from 3090 to 3790 ft displaying pervasive superimposed GR cycles with thicknesses of 10, 20, 50, and 200 ft (see “Symbols”), possibly indicating, respectively, precession index, obliquity, short orbital eccentricity, and long orbital eccentricity cycles.
Also intensifying through later Permian time was the Pangaean megamonsoon, repeatedly inferred from both modeling and data (e.g., Kutzbach and Gallimore, 1989; Parrish, 1993; M. Soreghan et al., 2002, 2018; Tabor and Montanez, 2002) but poorly constrained in terms of its evolution and drivers. Dust (loess) deposits capture an excellent archive of the monsoon, as well documented by work on the Quaternary monsoonal record archived in the Chinese Loess Plateau (e.g., Sun et al., 2006), and the Permian preserves the most voluminous dust deposits known in Earth's history (Soreghan et al., 2008b, 2015a). But the drivers behind this remarkable production and accumulation of dust and the effects of such an extreme (mineral-aerosol-rich) atmospheric composition remain unknown. The forcing(s) associated with atmospheric aerosols constitute one of the largest uncertainties in climate modeling from near to deep time (Schwartz and Andreae, 1996; NRC, 2011; Heavens et al., 2012; Lee et al., 2016). Continuous coring will enable us to tap the full extent of this dust record recovered in unambiguous stratigraphic superposition, assess extent of atmospheric dust loading, and use dust provenance (with, e.g., geochemistry and detrital zircon geochronology) to build a high-resolution archive of atmospheric circulation of unprecedented (tens of millions of years) duration. Because targeted dust deposits lie in both western and eastern Pangaea, we can assess spatiotemporal patterns of continental climate change, e.g., the nature and pace of proposed eastwardly progressing aridification (Tabor and Poulsen, 2008). Quantitative cyclostratigraphic analysis of high-quality well-log records as shown in Fig. 3 will enable assessment of astronomical forcing of tropical climate/hydroclimate through the Permian icehouse–greenhouse transition up to the end-Permian mass extinctions. The discovery of astronomical forcing signatures in continental western Pangaea will provide “floating” astrochronologies and opportunities to correlate the signatures with biostratigraphically constrained marine Paleotethyan counterparts described by Wu et al. (2013) and Fang et al. (2015, 2017) and with continental eastern Pangaea (Huang et al., 2020; Pfeifer et al., 2020b). These correlations will be enhanced greatly by adding paleomagnetic and (post-Kiaman) polarity data, achievable by virtue of obtaining continuous records in both western and eastern equatorial Pangaea.
2.3 The later Permian: extreme environmental and biotic records leading up to the Great Dying
The later Permian accommodates the final icehouse collapse and the transition to one of the most extreme greenhouse times known in Earth's history (Triassic), with the intercalated largest extinction known – the Permian–Triassic (P–T) extinction event. The P–T event has been linked in part to massive large igneous province (LIP) volcanism in Siberia (Siberian Traps), a region containing enormous volumes of carbon- and evaporite-rich strata, exacerbating the release of large amounts of greenhouse gases and halocarbons into the atmosphere (e.g., Svensen et al., 2009). Middle Permian–early Triassic red-bed-hosted halite records extreme continental conditions, such as hyperaridity, local air temperatures as high as 73 ∘C, and a diurnal temperature range up to 28 ∘C (Zambito and Benison, 2013), as well as extremely acid saline lakes and groundwaters with pH as low as 0 (Benison et al., 1998; Andeskie et al., 2018). New fluid inclusion methods have documented atmospheric O2 from Proterozoic halite (Blamey et al., 2016), suggesting that atmospheric O2 may also be analyzed from fluid inclusions in Permian halite; these types of analyses are uniquely enabled by coring the types of deposits preserved in the target regions. Much of the research on the run-up to the P–T crisis focuses on the marine record (e.g., Erwin, 1994; Wignall and Twitchett, 1996). In contrast, this proposed ICDP project will target high-resolution, continental successions sensitive to climatic and environmental conditions, including lacustrine, paleosol, and fine-grained eolian (e.g., loess) deposits, that we anticipate have the potential to preserve palynological as well as other records of paleoecological conditions and transitions. Physiological performance in modern tropical ectotherms is impacted by even small-magnitude climate warming, underscoring the vulnerability of low-latitude regions to climate change. Spanning the equatorial region west to east enables differentiation of local from regional-to-global changes in the continental record of the conditions leading to extinction and test hypotheses of a west–east lag in the loss of peri-Tethyan aquatic diversity and increased terrestrial diversity (Pardo et al., 2019). We anticipate the possibility that the targeted coring regions will enable elucidation of climatic and biotic changes through the middle and late Permian in particular, which are poorly documented.
2.4 Nature of the modern and fossil deep microbial biosphere
Discussion of questions on the deep microbial biosphere focused on both the microbes living today and those potentially preserved from Permian time – the latter representing a truly unique opportunity. The Anadarko Basin, for example, is the deepest sedimentary basin on the North American craton, with significant basinal fluid migration, documented by modern sulfide- and sulfur-rich springs such as Zodletone Spring (https://www.mindat.org/loc-305255.html, last access: 20 September 2020), which boosts a highly diverse and complex microbial ecosystem (Luo et al., 2005). Coring is essential to test the limits and nature of the deep biosphere here. Microbial life – potentially present in fluids or rock surfaces – might be driven by the redox transformation of abundant (trace) elements in the basin such as arsenic and iron (Amstaetter et al., 2010) or might utilize metabolic pathways associated with hydrocarbons (Hamamura et al., 2005; Foght et al., 2017).
As noted above, coring strata from the target basins hold the potential to enable unprecedented analyses of the fossil microbial biosphere, locked within fluid inclusions of Permian evaporites preserved exclusively at depth (e.g., Satterfield et al., 2005; Benison, 2019). These previous studies demonstrated that microbial life existed in the Permian at pH < 1, pH < 0, and pH < −1 – among the environments with the lowest pH and water activity known to host life (Benison, 2013). Several questions arise from these observations, such as whether the Permian microbes remain viable and whether relationships exist between the modern and Permian microbial biospheres, for which the host lithology has remained constant for nearly 300 Myr. Finally, such extreme, iron-rich, acidic conditions as those of these Permian red beds provide sedimentary analogs to past Martian surface conditions, including potential biosignatures. The Permian evaporites of Kansas preserved only in cores contain fossil microbes and organic compounds both as solid inclusions and within fluid inclusions (e.g., Benison, 2019). Iron oxide concretions in recent siliciclastic sediments associated with acid saline lakes in Western Australia contain microfossils (Farmer et al., 2009). Our proposed coring would provide reference comparison samples for sediments and rocks returned from Mars, with significant implications for astrobiology.
2.5 Auxiliary science
Finally, breakout groups discussed auxiliary science objectives that could be pursued in addition to the primary paleoclimatic/paleoenvironmental/microbial objectives. These objectives included red-bed sedimentology, induced seismicity, tectonics, geothermal energy, hydrology, and drilling engineering. Additionally, a fundamental advance will be the ability to build paleomagnetic and magnetostratigraphic reference sections for the Permian to help resolve long-standing paleogeographic enigmas with implications for paleoclimate. If we wish to advance paleoclimatic understanding and disentangle local from global effects, we must accurately locate the study areas in paleogeographic space. A viable approach to resolving the ongoing controversy of Pangaea A versus Pangaea B configurations (Fig. 1), for example, is by magnetic studies of long continuous cores at the target sites.
The prevalence of Permian red beds is renowned but puzzling; ideas for this geochemical anomaly have centered predominantly on a climatic control (Barrell, 1908; Walker, 1967, 1974; Dubiel and Smoot, 1994; Parrish, 1998), although Sheldon (2005) called into question whether red indicates any specific paleoclimatic parameters. The remarkable atmospheric oxygen levels perhaps played a role (e.g., Glasspool and Scott, 2010). More recent data on iron geochemistry of Permian loess from scattered sites throughout the western US reveal mysteriously high iron bioavailability (i.e., high values of highly reactive iron to total iron), with major implications for fertilization of primary productivity and thus carbon cycling (Sur et al., 2015; Sardar Abadi et al., 2020) during this interval, yet the origin of this reactivity remains enigmatic. Coring would enable assessment of iron reactivity in dust across vast stretches temporally and spatially: through the entire Permian, and across the tropical landmass, which would help address drivers for the reactivity and help address the origin of red beds.
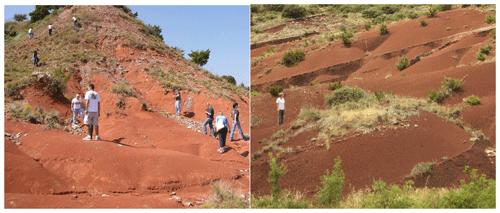
Figure 4Examples of the lower Permian paleo-loess of (left) the mid-continent US and (right) France. Left: the middle Permian Flowerpot Shale of the Anadarko Basin, western Oklahoma (Sweet et al., 2013). Right: the middle Permian Salagou Formation of the Lodève Basin, southern France (Pfeifer et al., 2016, 2020a, b).
The proposed sites capture rather unique tectonic settings, as both the Anadarko Basin and Permian basins of western–central Europe occupy regions either proximal to or atop (respectively) collapsed paleo-highlands of the greater Central Pangaean Mountains. Both received sediment from major orogenic belts of these systems: the Appalachian orogen in the case of the Anadarko Basin (e.g., Soreghan et al., 2019) and the Variscan core in the case of Permian basins of France (e.g., Pfeifer et al., 2016). Given the expected continuity of the target sections, it might be possible to constrain unroofing of the Appalachian and Variscan orogens using detrital zircon and apatite thermochronology. Preliminary work on a partial Permian section from the Lodève Basin (southern France) revealed remarkably high rates (1–17 mm yr −1) of exhumation in the Variscan orogen, comparable to present rates of Himalayan uplift (Pfeifer et al., 2016). Together with recent work that reveals mean Variscan paleoelevations of 3300±1000 m at ca. 300 Ma (Dusséaux, 2019), this has significant implications for understanding climatic–tectonic interactions.
The incidence of induced seismicity in Oklahoma (and many other regions, especially in regions exploited for hydrocarbon or geothermal energy) has increased significantly in the last decade and holds significant societal implications. In Oklahoma, most of the induced seismicity is linked to fluid injection associated with wastewater disposal or hydraulic fracturing (Chen et al., 2017; Ellsworth, 2013; Keranen and Weingarten, 2018; Langenbruch et al., 2018), where most of the wastewater is produced from unconventional shales that commonly include significant ratios of formation saltwater along with the hydrocarbons (Ellsworth, 2013). Globally, incidences of induced seismicity have been associated with fluid injection, hydrocarbon extraction, and geothermal energy, among many other causes (Foulger et al., 2018). Instrumentation of a borehole with fiber-optic cabling could enable distributed acoustic sensing of seismicity, strain, and auxiliary assessments of geothermal gradient, and shallow hydrogeology helpful for studying seismicity as well as a number of other studies. Observations of these parameters are relatively rare in the target region of Oklahoma. Finally, a fully cored borehole could be a compelling test facility for new drilling technology – specifically as a geothermal test well. Most oil and gas wells do not have the required size or depth required for clean energy development such as enhanced geothermal systems. In western Europe, Permian strata are a growing target for potential geothermal energy production; specific in situ measurements (e.g., hydraulic production test) and high-resolution lab investigations (petrophysics properties, small-scale fracturing, temperatures) would serve as a reference for future investigations in both the US and European sites.
Targeted sections preserve primarily continental records because the main science drivers center on paleoclimate and paleoenvironments. Between the western and eastern equatorial sites, lithologic compositions expected potentially include (predominantly) paleo-loess deposits and subordinate evaporites in lowland basinal sections (Fig. 4) but also mixed-lithology (carbonate–clastic) cyclothems of epeiric deposition (basal Permian) in basinal sites and possible ice-contact or pro-/peri-glacial deposits in upland-proximal sites. For the lowland sites, we are specifically targeting sections expected to consist predominantly (>80 %) of fine-grained sediments thought to comprise loess and dust trapped ultimately in lacustrine settings. Like lacustrine sediment, loess is considered the most comparable to marine sediment in recording continuous- or near-continuous deposition (Begét and Hawkins, 1989); accordingly, loess and lakes archive the highest-resolution continental archives for paleoclimatic and auxiliary reconstructions. Loess deposits in particular, which include intercalated paleosols, preserve up to millennial-scale records of continental climate, including atmospheric circulation (dust loading, wind direction, and strength from provenance and grain size) and precipitation (from magnetic susceptibility, e.g., M. Soreghan et al., 2014; Yang and Ding, 2013; Soreghan et al., 2015a; Maher, 2016) as well as – potentially – paleotemperature and atmospheric pCO2 in hosted paleosols (e.g., Tabor and Myers, 2015). Anisotropy of magnetic susceptibility in loess is well studied as an excellent potential for reconstructing wind directions and also intensity variations (e.g., Liu et al., 1999; Zhu et al., 2004; Nawrocki et al., 2006; Zhang et al., 2010). Eolian dust trapped by lacustrine or shallow marine environments holds the potential for organic preservation and thus associated organic geochemical and biomarker analyses. Evaporites preserve continental temperatures and brine compositions at temporal resolutions as fine as diurnal (e.g., Benison and Goldstein, 1999; Zambito and Benison, 2013). Cyclothems preserve an archive of glacial–interglacial variations from which we can potentially extract paleotemperature, ice-volume variations, and continental weathering flux (e.g., Elrick and Scott, 2010; Theiling et al., 2012; Elrick et al., 2013). Proglacial and associated deposits establish the former extent and nature of glaciation.
Thermochronology through clastic successions can reveal long-term denudation in these uplift-proximal regions, potentially shedding light on both tectonic and tectonic–climatic interactions (e.g., Reiners and Brandon, 2006). Many of the sedimentologic, geochemical, and fluid inclusion tools require pristine samples recoverable only by coring, and achievement of high-resolution temporal trends, especially those employing, e.g., quantitative cyclostratigraphy and magnetostratigraphy, demand continuous and stratigraphically complete sampling through sections with unambiguous stratigraphic superposition. Currently, we lack such data for the Permian; rather, climate-sensitive records are limited to spatially and temporally discrete sampling of mostly outcrops, with very few North American data in the later Permian (e.g., Tabor and Poulsen, 2008; atmospheric pCO2 data of Montañez et al., 2007, 2016). These studies suggest an increasing trend in pCO2 and equatorial aridity from the late Carboniferous to the middle Permian as well as substantial glacial–interglacial variability in both parameters from the middle Carboniferous to the early Permian.
The Anadarko Basin (Oklahoma, USA) and Paris Basin (France, western Europe) preserve what are likely the most complete records of the continental Permian on Earth and sample the antipodes of equatorial Pangaea. Furthermore, the partial preservation of paleo-upland regions in both the Wichita Uplift (Anadarko Basin system; Soreghan et al., 2012) and Variscan system (below the Brécy Basin; Beccaletto et al., 2015) presents the rare opportunity to access archives of moderate-paleoelevation sites; together with ongoing research positing high elevations (>3000 m; Dusséaux, 2019) and possible upland glaciation for the Variscan system, these localities are globally unique.
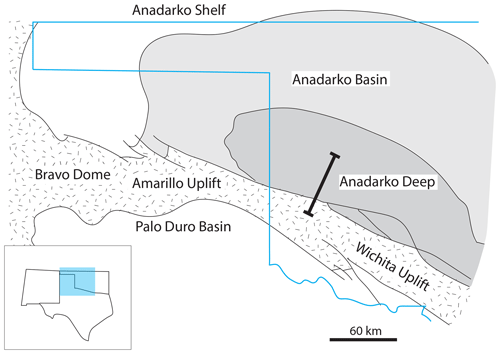
Figure 5Plan view image of the Anadarko Basin (modified from Hentz, 1994). The bold line denotes the approximate location of the 3D seismic line in Fig. 7. Note that both the Amarillo Uplift and Bravo dome (and much of the Wichita Uplift) are in the subsurface.
4.1 Western Pangaean site: Anadarko Basin (Oklahoma)
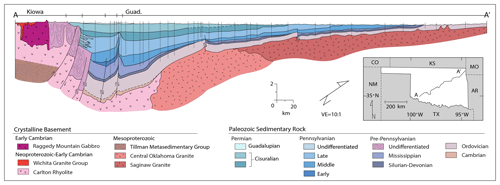
Figure 6Cross section of the Anadarko Basin, from the basin deep (SW) to the shelf (NE). Thin vertical lines are locations of the boreholes (logs) used for the interpretation. The seismic line (shown as “S” on the inset map) in Fig. 7 depicts (approximately) the boxed region. Cross section modified from Witt et al. (1971).
The Anadarko Basin (Fig. 5) of the southern mid-continental US is the deepest sedimentary basin on the North American craton, owing to a dual history of Neoproterozoic-Cambrian (failed) rifting followed 300 Myr later by late Paleozoic compressional deformation associated with western Pangaean assembly (Johnson, 1988). This basin represents the southern limit of a widespread subsiding region during Permian time that extended across mid-continental North America – a largely endorheic basin akin in scale and character to the greater Lake Chad region of modern Africa. The sedimentary record in the Anadarko Basin is especially complete for the late Carboniferous–Permian, with preservation of ∼2000 m of Permian strata alone (Soreghan et al., 2012; Fig. 6) comprising predominantly continental (paleo-loess/dust, lacustrine, and evaporite) deposits, with (basal) epeiric cyclothems of a mixed carbonate–clastic character (Johnson, 1988). Remarkably, the Permian subsidence event affected both the basin and the adjacent uplift (Wichita Uplift), resulting in preservation of a Permian landscape (Soreghan et al., 2012) and burial of upland strata in the subsurface along the southern basin margin. Industry seismic data are accessible to guide drill site locations (cf. Fig. 7).
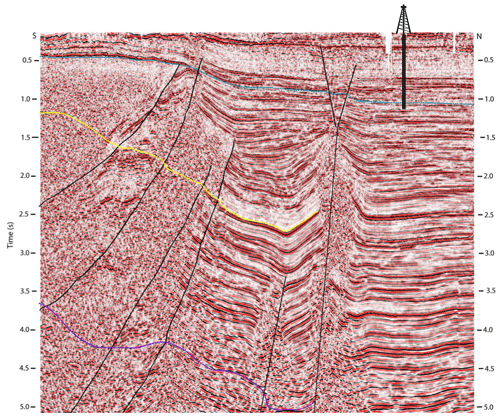
Figure 7Vertical time slice from a 3D seismic volume acquired across the Frontal Fault zone of the Wichita–Anadarko system, extending from the (subsurface) Wichita Uplift (left) into the deep Anadarko Basin (right). See Fig. 6 for the approximate location of the line (line length ∼60 km). Vertical scale shows two-way travel time (seconds). Stratal markers as follows: base Pennsylvanian – yellow, base Permian – blue. From Rondot (2009). A potential (basinal) drill site is indicated with the derrick symbol.
4.2 Eastern Pangaean site(s): European target(s)
The Deep Dust 2.0 workshop focused specifically on a comprehensive assessment of Permian basins in western and central Europe. Participants converged on prioritizing a reference section that could reasonably be expected to house a continuous section of predominantly fine-grained continental facies that includes the C–P boundary and approaches as closely as possible the P–T boundary. The only area in western Europe containing the P–T boundary occurs in the central part of the Germanic Basin (Bourquin et al., 2011), and the correlation with the Paris Basin is well established (e.g., Bourquin et al., 2006, 2009). Moreover, the C–P boundary is well defined and dated in nearby outcrops and subsurface data (Ducassou et al., 2019; Pellenard et al., 2017; Mercuzot et al., 2020; Mercuzot, 2020). A number of basins in Germany contain parts of the Permian, with extensively cored sections, but the common occurrence of coarse-grained, high-energy facies (e.g., inferred alluvial-fan, fluvial, volcaniclastic) and lack of a single site traversing the entire (or nearly entire) section are problematic from the perspective of the primary science drivers for the Deep Dust project. Other criteria considered in narrowing the selection included availability of seismic data (constrained by boreholes), thicknesses of target and overburden, and expected dating potential (e.g., by ashes and/or biostratigraphy). Ultimately, the southwestern Paris Basin emerged as the best location for a reference section. However, participants were keen to consider a drilling program that could include additional localities in a staged approach. The Permian of eastern equatorial Pangaea (France) is partially exposed in dismembered rift basins of the Variscan–Hercynian orogenic belt of central and southern France, such as the Lodève Basin (Fig. 8) that formed as a result of orogenic collapse (Burg et al., 1994; Praeg, 2004). These basins preserve a sedimentary succession analogous to that of the Anadarko Basin, with drab-hued carbonaceous upper Carboniferous strata yielding upsection to fine-grained red-bed lacustrine, eolian, and rare evaporitic sediments through the Permian (e.g., Pochat and Van Den Driessche, 2011), including extensive loess deposits (Pfeifer et al., 2016, 2020a). In the Paris Basin, numerous late Paleozoic “sub-basins” have been recognized since the 1960s but lie beneath the Ceno-Mesozoic cover. The southwestern Paris Basin in particular remains virtually unexplored with no core except for a 110 m core of Permian red beds (Juncal et al., 2018) and three wells reaching a maximum of 1600 m in the upper Carboniferous to upper Permian section (Bertray, Brécy, and Saint-Georges-sur-Moulon) with available well-log data (gamma ray, sonic) and cutting descriptions. Recent pre-stack migration processing reprocessing of vintage seismic data reveals a significant Permian section here (Fig. 10). The Permian section extends over an area of several thousand km2, with thicknesses reaching 3000 m (Beccaletto et al., 2015; Figs. 9, 10).
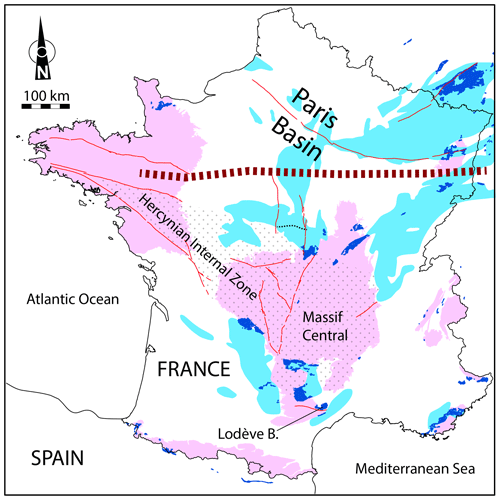
Figure 8Top – simplified geology of France. Dark blue regions are basins with Permian strata exposed (e.g., Lodève), whereas light blue regions denote Permian strata buried by Mesozoic overburden. The bold dotted line crossing the E–W span of the Paris Basin is the location of the cross-section line in Fig. 9. The fine dotted line in the southern–central part of the basin is the location of the seismic line in Fig. 10 (modified from Beccaletto et al., 2015).
Permian strata of the southwestern Paris Basin accumulated “above” the internal zone and suture of the Variscan belt, south of the Variscan front during the late-to-post orogenic extensional processes (Faure, 1995; Baptiste et al., 2016). These basins formed after the main Variscan deformation and thus the Permian strata remain virtually undisturbed by Variscan orogenesis. The post-orogenic subsidence (from tectonic to thermal) during the late Paleozoic enabled preservation of a nearly complete stratigraphic succession from late Carboniferous to late Permian time, with an unconformity at the Permian–Triassic boundary (as is the case across western–central Europe; e.g., Bourquin et al., 2011). Strata of the southwestern Paris Basin accumulated during two successive tectonic phases (Beccaletto et al., 2015).
-
An initial period of opening of the (Arpheuilles, Contres, and Brécy) basins, during which Stephanian conglomeratic/coal facies accumulated under a strong structural control (normal- and strike-slip faulting, with development of a clastic wedge); this represents about 20 % of the (Stephanian)–Permian section.
-
Subsequent Permian and pre-Triassic tectonic activity (northwest–southeast-oriented strike–slip faulting in the Arpheuilles Basin, uplift of the margins of the three basins), resulting in tectonic subsidence and associated sedimentation in these basins. The Permian strata here consist mainly of mudstone or silty mudstone, with rare thin interbeds of sandy, carbonate, intra-clastic conglomeratic, or evaporitic facies. Occurrences of fine to coarse sandstone occur in the top interval. These lithologies are analogous to those in neighboring basins, where they are interpreted as restricted lacustrine to localized fluvial facies and are analogous to those inferred for the Anadarko Basin of Oklahoma.
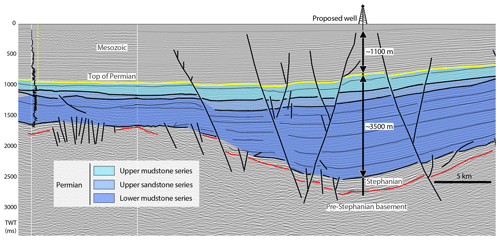
Figure 10Southwestern Paris Basin (Brécy Basin) seismic line (line of section indicated by fine dotted line in the southern Paris Basin in Fig. 8). Seismic interpretation of lithologies and estimation of thicknesses from the main depocenter in the Brécy area, with a potential drill site indicated by the derrick symbol.
Thus, although the uppermost Carboniferous–lower Permian strata exist in isolated basins, the rest of the Permian sedimentary section accumulated across a broad region as demonstrated by the reinterpretation of newly reprocessed seismic lines (Fig. 10). As these basins were later covered by Triassic strata (Fig. 9), the Permo-Carboniferous section was essentially protected from Meso-Cenozoic tectonic uplift and subsequent erosion. Importantly, this same situation does not hold for the strata now exposed in regions such as the Lodève Basin. The Permian of subsurface basins such as those beneath the southwestern Paris Basin have been affected by a slight pre-Triassic tilting and by Oligocene brittle normal faulting (represented by nearly vertical faults in Fig. 10), without major tectonic perturbation. Other northern European basins offer less complete and partly marine stratigraphic sections for this interval, owing to slower rates of subsidence and the influence of the Zechstein Sea (e.g., Doornenbal and Stevenson, 2010).
We provisionally propose the southwestern Paris Basin as our coring target to capture a continuous lowland record in eastern equatorial Pangaea. We recommend a coring target in what is projected to be the most stratigraphically complete section: in the Brécy (sub)basin, where the Permian reaches a thickness of ∼3000 m, beneath a Mesozoic cover of ∼1000 m (Figs. 9, 10).
Intact and complete continental stratal records from the mid-Permian through Triassic of Pangaea are rare (in both outcrop and standard cores). Within the Anadarko Basin, outcrops are stratigraphically and lithologically incomplete due to intercalated halite in parts of the section, which dissolves at and near the surface (Benison et al., 2015). Furthermore, the gentle dip across a region with minimal relief creates limited outcrop opportunities. Although the Anadarko Basin hosts hundreds of thousands of boreholes owing to nearly a century of hydrocarbon extraction, minimal core exists of the Permian owing to its status as overburden. However, the rare cores that have been recovered yield a wealth of high-resolution paleoenvironmental, paleoclimatological, and microbiological data about Pangaea (e.g., Benison et al., 1998; Zambito and Benison, 2013; Foster et al., 2014; M. Soreghan et al., 2018; Benison, 2019; Andeskie and Benison, 2020). Similarly, although the Paris Basin has been a target for resources preserved in especially the Mesozoic section, no extensive Permian core exists. Here, the Permian is reachable exclusively through subsurface coring.
Coring will enable acquisition of long, continuous records through even friable lithologies, with the unambiguous stratigraphic superposition needed to construct permanent reference sections for the Permian. It will also enable exploration of sediment preserved atop buried paleouplands. The importance of this time period, encompassing biotic crises such as the end-Guadalupian biotic event and major climate change, combined with the exceptional preservation of rocks by purposeful and well-planned coring, makes a compelling case for scientific drilling (Soreghan et al., 2014). Finally, continuous coring of stratigraphically unambiguous sections will enable application of comprehensive paleomagnetic and magnetostratigraphic studies critical to resolving arguably the most fundamental aspect of the Permian world: which geographic reconstruction (Fig. 1) is accurate?
Permian strata of the Anadarko Basin have been dated with invertebrate as well as vertebrate fauna, palynology, chemostratigraphy, magnetostratigraphy, and geochronology (tephras), with much work occurring relatively recently (e.g., Denison et al., 1998; Steiner, 2006; Tabor et al., 2011; Geissman et al., 2012; Foster, 2013; Tian et al., 2020). The strata in Permian basins of France accumulated in close proximity to major volcanic centers and thus contain abundant, dateable ash beds (e.g., Bruguier et al., 2003; Michel et al., 2015). We envision greatly refining age models through the Permian in both regions using a combination of the following approaches.
-
Geochronology: ash beds are well known throughout the Carboniferous–Permian section of, e.g., the Lodève Basin (Michel et al., 2015), southern Paris Basin (Ducassou et al., 2019), Autun Basin (Pellenard et al., 2017), and associated Carboniferous–Permian basins of France and adjoining regions, and these should be especially visible in the core. Permian volcanism was farther removed from the Anadarko Basin, but tephras (likely from Permian arc(s) of northwestern Mexico) are well recognized in the youngest Permian strata here (Tabor et al., 2011; Geissman et al., 2012) and have been long recognized by industry geologists in the Upper Pennsylvanian and Permian sections of the nearby Permian Basin in western Texas (personal communication, E. Kvale, 2015; S. Ruppel, 2016; Tian et al., 2020). High-resolution chemical abrasion ID-TIMS zircon geochronology can be employed to provide essential absolute ages, aid in correlations, and constrain sedimentation rates. Additionally, in regions distal to (but downwind of) volcanic eruption centers, detrital zircon geochronology can augment dating by recovering a cluster of the youngest zircons to provide a maximum age of deposition (e.g., Fan et al., 2015); detrital zircons are abundant in fine-grained Permian strata of both the Anadarko and French basins (Sweet et al., 2013; Foster et al., 2014; Pfeifer et al., 2016).
-
Magnetostratigraphy: although the Permian encompasses the Kiaman Superchron, spanning from ∼320 Ma (mid-Bashkirian) to ∼265 Ma (late Wordian), the Permian of both the Anadarko and French Permian basins captures the termination of the superchron and the subsequent record of reversal stratigraphy (Steiner, 2006; Foster et al., 2014; Evans et al., 2014), recognizable even at these relatively low paleolatitudes. Recovery of continuous core with unambiguous stratigraphic superposition is critical for magnetostratigraphic efforts.
-
Chemostratigraphy: Sr isotopes have been applied to carbonate and sulfate-rich strata in the Permian of the Anadarko Basin (Denison et al., 1998), but the potential exists to build on this, particularly because of the dramatic decline in the 87Sr∕86Sr through the proposed study interval. In addition, carbon isotope stratigraphy of early diagenetic dolomitic cements in the upper Permian and Permo-Triassic boundary strata from the nearby Palo Duro Basin suggest that carbon isotope stratigraphy may be a viable means of stratigraphic correlation for later Permian strata (Tabor et al., 2011).
-
Paleontology: for the continental sections, paleobotanical specimen data can be quite useful and can be preserved in red beds. For example, for the Anadarko Basin, regional trends in the appearance and relative abundance of marker taxa from both wetland and seasonally dry assemblages can be tied to well-characterized sections in northern–central Texas and central New Mexico, thereby facilitating an understanding of regional vegetational patterns (e.g., DiMichele et al., 2006). The same is true for the pollen assemblages these plants produced. Pollen and spores (palynomorphs) are generally poorly preserved in red beds but common in non-oxidized sediments, including the Permian of the Anadarko Basin (e.g., Wilson, 1962), and can occur in evaporites intercalated within the red beds. The valves of Conchostracans (clam shrimps) are also well known and zoned from Permian red beds (Scholze and Schneider, 2015). For the limestone-bearing epeiric strata of the lowermost section in the Anadarko and French Permian basins, conodonts and fusulinids are abundant and well zoned at cyclothem-scale (substage) resolution. Sedimentological information, such as grain size (silts and clays) and sediment color (darker gray), can be used to identify the most promising facies for palynological analysis.
-
Cyclostratigraphy: the emerging chronostratigraphic power of cyclostratigraphy will likely play an important role in dating the cores. Astronomical forcing is global, and so astronomically forced cyclostratigraphic patterns should be correlatable from marine to continental facies, in the same way that, for example, the Quaternary Chinese Loess Plateau magnetic susceptibility record (Fig. 12 in Ding et al., 2002) and the Lake Baikal biosilica record (Fig. 3 in Williams et al., 1997) correlate with the marine δ18O record. For much (if not all) of the time coverage anticipated for these cores, there is either existing marine cyclostratigraphy tied to conodont zones and/or radioisotope dating (Wu et al., 2013, 2019; Fang et al., 2015, 2017), which makes this dating approach possible.
To tackle the problem of constructing a complete geochronology, proxy stratigraphic series constructed from the cores will be analyzed for plausible sediment accumulation rates that convert the proxy sequences into a time series with significant astronomical frequencies. This is the goal of the objective methods “average spectral misfit” (Meyers and Sageman, 2007) and “time optimization” (TimeOpt; Meyers, 2015, 2019). Successful ASM or TimeOpt applications can be used to extract the orbital eccentricity signal from the proxy sequences, which then can be matched to similarly processed marine cyclostratigraphy using statistical correlation methods such as Match (Lisiecki and Lisiecki, 2002) or HMM-Match (Lin et al., 2014). The results of these statistical correlations can be used to project marine biostratigraphic (conodont, fusulinid) zones, as well as other age data, into the core sections.
The events of the Permian capture profoundly extreme swings in Earth system behavior, justifying a focus on paleoenvironmental conditions for this project, but with strong auxiliary science objectives. Two sites are admittedly inadequate to capture global conditions, but the proposed initial sites in the Anadarko and Paris basins offer unrivaled opportunities to establish stratigraphically complete global reference sections spanning equatorial Pangaea, to which various outcrop sections could be tied for building an integrated record. Abundant opportunities exist for science outreach and education associated with the remarkable events of the Permian – the icehouse-to-greenhouse transition, Great Dying, mega dust bowl and megamonsoon, Mars analog conditions, and the Permian megafauna of the equatorial region, in addition to the auxiliary science topics.
No data sets were used in this article.
The supplement related to this article is available online at: https://doi.org/10.5194/sd-28-93-2020-supplement.
Steve Adams (USA), Jennifer Auchter (USA), Cédric Bailly (France), Lucas Pinto Heckert Bastos (Brazil), Sietske Batenburg (France), Laurent Beccaletto (France), Heather Bedle (USA), Kathy Benison (USA), Alicia Bonar (USA), Didier Bonijoly (France), Sylvie Bourquin (France), Brett Carpenter (USA), Teodoriu Catalin (USA), Ying Cui (USA), Anne-Christine Da Silva (Belgium), Anne-Laure Decombeix (France), Jose. B. Diez (Spain), David Duarte (USA), Shannon Dulin (USA), Steve Dworkin (USA), Majie Fan (USA), Chris Fielding (USA), Tracy Frank (USA), Georg Feulner (Germany), Frédéric Fluteau (France), Sylvain Garrel (USA), Aude Gebelin (GB), Ute Gebhardt (Germany), Svetoslav Georgiev (USA), Charles Gilbert (USA), Martha-Elisabeth Gibson (GB), Annette E. Götz (Germany), Stéphane Guillot (France), Natsuko Hamamura (Japan), Mike Hamilton (Canada), Uli Harms (Germany), Nick Heavens (USA), Christoph Heubeck (Germany), Linda Hinnov (USA), Adam K. Huttenlocker (USA), Libby R. W. Ives (USA), Ken Johnson (USA), Sue Kairo (USA), Lee Krumholz (USA), Alex Lay (USA), Richard Lupia (USA), Andy Madden (USA), Joe Mason (USA), Austin McGlannan (USA), Eduardo Luiz Menozzo Da Rosa (USA), Mathilde Mercuzot (France), Yuki Morono (Japan), Andrea Moscariello (Switzerland), Amy Myrbo (USA), Pierre Nehlig (France), Anders Noren (USA), Hendrik Nowak (Italy), Francisca Oboh-Ikuenobe (USA), Silvia Omodeo-Sale (Switzerland), Jeremy Owens (USA), Damien Pas (Belgium), Kathryn N. Pauls (USA), Lily Pfeifer (USA), Pierre Pellenard (France), Isabella Ploch (Poland), Stéphane Pochat (France), Marc Poujol (France), Nathan M. Rabideaux (USA), Jahandar Ramezani (USA), Troy Rasbury (USA), Zeev Reches (USA), Jean-Baptiste Regnet (France), Dean Richmond (USA), Philippe Robion (France), Lara Rodriguez-Delgado (USA), Alastair Ruffell (GB), Mehrdad Sardar Abadi (USA), Johann Schnyder (France), Lynn Soreghan (USA), Amalia Spina (Italy), Holly Stein (USA), Alisan Sweet (USA), Ryan Totten (USA), Arne Ulfers (Germany), Huaichun Wu (China), Chenliang Wu (China), Wan Yang (USA), Molly Yunker (USA), Richard Yuretich (USA), Jay Zambito (USA), Christian Zeeden (Germany).
GSS convened the workshop in Oklahoma, drafted the manuscript, and conducted revisions. LB and SB convened the workshop in Paris and contributed text and figures relevant to the Paris Basin and European geology. KCB, GF, NM, MH, NGH, LH, AH, CL, LSP, SP, MSA, and JZ contributed text and/or figure content. Participants at both workshops contributed intellectual input.
The authors declare that they have no conflict of interest.
We thank ICDP for workshop funding of the Deep Dust project and all participants for constructive input and enthusiasm. We especially thank Uli Harms for continued encouragement and constructive suggestions and PhD student Lily Pfeifer for essential help in organizing the Norman workshop. Gerilyn S. (Lynn) Soreghan also thanks staff from the OU School of Geosciences (especially Ashley Tullius), faculty of the OU History of Science Department (Kerry Magruder) for additional logistical help, and Terry Nitzel for land access. We thank the reviewers for many constructive comments on an earlier version of this paper. Those interested in participating in Deep Dust are invited to contact the authors. We thank Enervest (S. Adams), SEI, and CGG for seismic images used during the workshop.
This research has been supported by the ICDP (DeepDust2019 grant).
This paper was edited by Jan Behrmann and reviewed by Flavio Anselmetti and Paul Olsen.
Alroy, A., Aberhan, M., Bottjer, D. J., Foote, M., Fürsich, F. T., Harries, P. J., Hendy, A. J. W., Holland, S. M., Ivany, L. C., Kiessling, W., Kosnik, M. A., Marshall, C. R., McGowan, A. J., Miller, A. I., Olszewski, T. D., Patzkowsky, M. E., Peters, S. E., Villier, L., Wagner, P. J., Bonuso, N., Borkow, P. S., Brenneis, B., Clapham, M. E., Fall, L. M., Ferguson, C. A., Hanson, V. L., Krug, A. Z., Layou, K. M., Leckey, E. H., Nürnberg, S., Powers, C. M., Sessa, J. A., Simpson, C., Tomašových, A., and Visaggi, C. C.: Phanerozoic trends in the global diversity of marine invertebrates, Science, 321, 97–100, https://doi.org/10.1126/science.1156963, 2008.
Amstaetter, K., Borch, T., Larese-Casanova, P., and Kappler, A.: Redox transformation of arsenic by Fe(II)-activated goethite (α-FeOOH), Environ. Sci. Technol., 44, 102–108, 2010.
Andeskie, A. S. and Benison, K. C.: Using sedimentology to address the marine or continental origin of the Permian Hutchinson Salt Member of Kansas, Sedimentology, 67, 882–896, 2020.
Andeskie, A. S., Benison, K. C., Eichenlaub, L. A., and Raine, R.: Acid-saline-lake systems of the Triassic Mercia Mudstone Group, County Antrim, Northern Ireland, J. Sediment. Res., 88, 385–398, 2018.
Baptiste, J., Martelet, G., Faure, M., Beccaletto, L., Reninger, P.-A., Perrin, J., and Chen, Y.: Mapping of a buried basement combining aeromagnetic, gravity and petrophysical data: The substratum of southwest Paris Basin, France, Tectonophysics, 683, 333–348, 2016.
Barrell, J.: Relations between climate and terrestrial deposits, continued, J. Geol., 16, 255–295, 1908.
Beccaletto, L., Capar, L., Serrano, O., and Marc, S.: Structural evolution and sedimentary record of the Stephano-Permian basins occurring beneath the Mesozoic sedimentary cover in the southwestern Paris basin (France), Bull. Soc. Géol. France, 186, 429–450, 2015.
Becq-Giraudon, J.-F., Montenat, C., and Van Den Driessche, J.: Hercynian high-altitude phenomena in the French Massif Central, tectonic implications: Palaeogeogr. Palaeocl., 122, 227–241, 1996.
Begét, J. E. and Hawkins, D. B.: Influence of orbital parameters on Pleistocene loess deposition in central Alaska, Nature, 337, 151–153, 1989.
Benison, K. C. and Goldstein, R. H.: Permian paleoclimate data from fluid inclusions in halite, Chem. Geol., 154, 113–132, 1999.
Benison, K. C.: Acid saline fluid inclusions: Examples from modern and Permian extreme lake systems, Geofluids, 13, 579–593, https://doi.org/10.1111/gfl.12053, 2013.
Benison, K. C.: How to search for life in Martian chemical sediments and their fluid and solid inclusions using petrographic and spectroscopic methods, Front. Environ. Sci., 7, 108, https://doi.org/10.3389/fenvs.2019.00108, 2019.
Benison, K. C., Goldstein, R. H., Wopenka, B., Burruss, R. C., and Pasteris, J. D.: Extremely acid Permian lakes and ground waters in North America, Nature, 392, 911–914, 1998.
Benison, K. C., Zambito, J. J., and Knapp, J. P.: Contrasting siliciclastic-evaporite strata in subsurface and outcrop: An example from the Permian Nippewalla Group of Kansas, USA, J. Sediment. Res., 85, 626–645, https://doi.org/10.2110/jsr.2015.43, 2015.
Berner, R. A.: GEOCARBSULF – A combined model for Phanerozoic atmospheric O2 and CO2, Geochim. Cosmochim. Ac., 70, 5653–5664, 2006.
Berner, R. A.: The long-term carbon cycle, fossil fuels and atmospheric composition, Nature, 426, 323–326, 2003.
Blamey, N. J. F., Brand, U., Parnell, J., Spear, N., Lecuyer, C., Benison, K. C., Meng, F., and Ni, P.: Paradigm shift in determining Neoproterozoic atmospheric oxygen, Geology, 44, 651–654, 2016.
Bourquin, S., Bercovici, A., López-Gómez, J., Diez, J. B., Broutin, J., Ronchi, A., Durand, M., Arche, A., Linol, B., and Amour, F.: The Permian-Triassic transition and the beginning of the Mesozoic sedimentation at the Western peri-Tethyan domain scale: palaeogeographic maps and geodynamic implications, Palaeogeogr. Palaeocl., 299, 265–280, 2011.
Bourquin, S., Guillocheau, F., and Péron, S.: Braided river within an arid alluvial plain (example from the early Triassic, western German Basin): criteria of recognition and expression of stratigraphic cycles, Sedimentology, 56, 2235–2264, 2009.
Bourquin, S., Peron, S., and Durand, M.: Lower Triassic sequence stratigraphy of the western part of the Germanic Basin (west of Black Forest): fluvial system evolution through time and space, Sediment. Geol., 186, 187–211, 2006.
Bruguier, O., Becq-Giraudon, J. F., Champenois, M., Deloule, E., Ludden, J., and Mangin, D.: Application of in situ zircon geochronology and accessory phase chemistry to constraining basin development during post-collisional extension: a case study from the French Massif Central, Chem. Geol., 201, 319–336, https://doi.org/10.1016/j.chemgeo.2003.08.005, 2003.
Burg, J.-P., Van Den Driessche, J., and Brun, J.-P.: Syn- to post thickening extension in the Variscan belt of western Europe: modes and structural consequences, Géologie de la France, 3, 33–51, 1994.
Cao, W., Williams, S., Flament, N., Zahirovic, S., Scotese, C., and Müller, R.: Palaeolatitudinal distribution of lithologic indicators of climate in a palaeogeographic framework, Geol. Mag., 156, 331–354, https://doi.org/10.1017/S0016756818000110, 2019.
Chen, X., Nakata, N., Pennington, C., Haffener, J., Chang, J. C., and He, X.: The Pawnee earthquake as a result of the interplay among injection, faults and foreshocks, Sci. Rep., 7, 1–18, https://doi.org/10.1038/s41598-017-04992-z, 2017.
Chamberlin, T. C. and Salisbury, R. D.: Geology – Vol II Earth History, Henry Holt and Company, New York, 1905.
Champagnac, J.-D., Schluneggar, F., Norton, K., von Blanckenburg, F., Abbuhl, L. M., and Schwab, M.: Erosion-driven uplift of the modern Central Alps, Tectonophysics, 474, 236–249, https://doi.org/10.1016/j.tecto.2009.02.024, 2009.
Chen, J., Montañez, I. P., Qi, Y., Shen, S., and Wang, X.: Strontium and carbon isotopic evidence for decoupling of pCO2 from continental weathering at the apex of the late Paleozoic glaciation, Geology, 46, 395–398, 2018.
Cleal, C. J. and Thomas, B. A.: Palaeozoic tropical rainforests and their effect on global climates: is the past the key to the present?, Geobiology, 3, 13–31, 2005.
Denison, R. E., Kirkland, D. W., and Evans, R.: Using strontium isotopes to determine the age and origin of gypsum and anhydrite beds, J. Geol., 106, 1–17, 1998.
DiMichele, W. A., Tabor, N. J., Chaney, D. S., and Nelson, W. J.: From wetlands to wet spots: environmental tracking and the fate of Carboniferous elements in Early Permian tropical floras, in: Wetlands through time, edited by: Greb, S. F. and DiMichele, W. A., Geol. Soc. Am. Spec. Pap., 399, 223–248, 2006.
Ding, Z. L., Derbyshire, E., Yang, S. L., Yu, Z. W., Xiong, S. F., and Liu, T. S.: Stacked 2.6-Ma grain size record from the Chinese loess based on five sections and correlation with the deep-sea ∂18O record, Paleoceanography, 17, 5-1–5-21, https://doi.org/10.1029/2001PA000725, 2002.
Domeier, M. and Torsvik, T. H.: Plate tectonics in the late Paleozoic: Geosci. Front., 5, 303–350, https://doi.org/10.1016/j.gsf.2014.01.002, 2014.
Doornenbal, J. C. and Stevenson, A. G. (Eds.): Petroleum Geological Atlas of the Southern Permian Basin Area. Houten, The Netherlands, EAGE, p. 342, 2010.
Dubiel, R. F. and Smoot, J. P.: Criteria for interpreting paleoclimate from red beds – a tool for Pangean reconstructions, in: Pangea: Global Environments and Resources, edited by: Embry, A. F., Beauchamp, B., and Glass, B. J., Canad. Soc. Petrol. Geol. Mem., 17, 295–310, 1994.
Ducassou, C., Mercuzot, M., Bourquin, S., Rossignol, C., Beccaletto, L., Pierson-Wickmann, A. C., Pellenard, P., Poujol, M., and Hue, C.: Sedimentology and U-Pb dating of Carboniferous to Permian continental series of the northern Massif Central (France): local palaeogeographic evolution and larger scale correlations, Palaeogeogr. Palaeocl., 533, 109228, https://doi.org/10.1016/j.palaeo.2019.06.001, 2019.
Dusséaux, C.: Topographic reconstructions of the Variscan belt of Western Europe through the study of fossil hydrothermal systems, PhD Thesis, University of Plymouth, 2019.
EL Hadi, H., Simancas, J. F., Tahiri, A., González-Lodeiro, F., Azor, A., and Martínez-Poyatos, D.: Comparative review of the Variscan granitoids of Morocco and Iberia: proposal of a broad zonation, Geodin. Acta, 19, 103–116, 2006.
Ellsworth, W. L.: Injection-induced earthquakes, Science, 341, https://doi.org/10.1126/science.1225942, 2013.
Elrick, M. and Scott, L. A.: Carbon and oxygen isotope evidence for high-frequency (104–105 yr) and My-scale glacio-eustasy in Middle Pennsylvanian cyclic carbonates (Gray Mesa Formation), central New Mexico, Palaeogeogr. Palaeocl., 285, 307–320, 2010.
Elrick, M., Reardon, D., Labor, W., Martin, J., Desrochers, A., and Pope, M.: Orbital-scale climate change and glacioeustasy during the Early Late Ordovician (Pre-Hirnantian) determined from ∂18O values in marine apatite, Geology, 41, 775–778, https://doi.org/10.1130/G34363.1, 2013.
Ernst, R. E. and Buchan, K. L.: Large mafic magmatic events through time and links to mantle-plume heads, in: Mantle Plumes: Their Identification Through Time, edited by: Ernst, R. E. and Buchan, K. L., Boulder, Colorado, Geol. Soc. Amer. Spec. Pap., 352, 483–575, 2001.
Erwin, D. H.: The Permo–Triassic extinction, Nature, 367, 231–236, 1994.
Erwin, D. H.: Extinction! How Life Nearly Ended 250 Million Years ago: Princeton University Press, 2006.
Evans, D. A. D.: A fundamental Precambrian-Phanerozoic shift in Earth's glacial style?, Tectonophysics, 375, 353–385, 2003.
Evans, M. E., Pavlov, V., Veselovsky, R., and Fetisova, A.: Late Permian paleomagnetic results from the Lodève, Le Luc, and Bas-Argens Basins (southern France): Magnetostratigraphy and geomagnetic field morphology, Phys. Earth Planet. Inter., 237, 18–24, https://doi.org/10.1016/j.pepi.2014.09.002, 2014.
Falcon-Lang, H. J. and DiMichele, W. A.: What happened to the coal forests during Pennsylvanian glacial phases?, Palaios, 25, 611–617, 2010.
Fang, Q., Wu, H. C., Hinnov, L. A., Jing, X. C., Wang, X. L., Yang, T. S., Li, H. Y., and Zhang, S. H.: Astronomical cycles of Middle Permian Maokou Formation in South China and their implications for sequence stratigraphy and paleoclimate, Palaeogeogr. Palaeocl., 474, 130–139, https://doi.org/10.1016/j.palaeo.2016.07.037, 2017.
Fang, Q., Wu, H., Hinnov, L. A., Jing, X., Wang, X., and Jiang, Q.: Geological evidence for the chaotic behavior of the planets and its constraints on the third order eustatic sequences at the end of the Late Paleozoic Ice Age, Palaeogeogr. Palaeocl., 440, 848–859, https://doi.org/10.1016/j.palaeo.2015.10.014, 2015.
Farmer, J. D., Bell III., J. F., Benison, K. C., Boynton, W. V., Cady, S. L., Ferris, F. G., MacPherson, D., Race, M. S., Thiemens, M. H., and Wadhwa, M.: Assessment of planetary protection requirements for Mars sample return missions, Space Studies Board, National Research Council, National Academy Press, Washington, D.C., 80 pp., 2009.
Faure, M.: Late orogenic Carboniferous extensions in the Variscan French Massif Central, Tectonics, 14, 132–153, 1995.
Feulner, G.: Formation of most of our coal brought Earth close to global glaciation, P. Natl. Acad. Sci. USA, 114, 11333–11337, https://doi.org/10.1073/pnas.1712062114, 2017.
Fielding, C. R., Frank, T. D., and Isbell, J. L.: The late Paleozoic ice age – a review of current understanding and synthesis of global climate patterns, 441, 343–354, 2008.
Foght, J. M., Gieg, L. M., and Siddique, T.: The microbiology of oil sands tailings: past, present, future, FEMS Microbiol. Ecol., 93, fix034, https://doi.org/10.1093/femsec/fix034, 2017.
Foster, G. L., Royer, D. L., and Lunt, D. J.: Future climate forcing potentially without precedent in the last 420 million years, Nat. Commun., 8, 14845, https://doi.org/10.1038/ncomms14845, 2017.
Foster, T. M., Soreghan, G. S., Soreghan, M. J., Benison, K. C., and Elmore, R. D.: Climatic and paleogeographic significance of eolian sediment in the Middle Permian Dog Creek Shale (Midcontinent U.S.). Palaeogeogr. Palaeocl., 402, 12–29, https://doi.org/10.1016/j.palaeo.2014.02.031, 2014.
Foulger, G. R., Wilson, M. P., Gluyas, J. G., Julian, B. R., and Davies, R. J.: Global review of human-induced earthquakes, Earth Sci. Rev., 178, 438–514. https://doi.org/10.1016/j.earscirev.2017.07.008, 2018.
Gastaldo, R., DiMichele, W., and Pfefferkorn, H.: Out of the icehouse into the greenhouse-A late Paleozoic analog for modern global vegetational change, GSA Today, 6, 1–7, 1996.
Geissman, J. W., Renne, P., Mitchell III., W. S., and Tabor, N. J.: Integrated magnetostratigraphy and geochronology of the “Ochoan” Quartermaster Formation of north Texas, Abstracts with Programs, Geol. Soc. Amer., 44, 29, 2012.
Giles, J. M., Soreghan, M. J., Benison, K. C., Soreghan, G. S., and Hasiotis, S. T.: Lakes, loess, and paleosols In the Permian Wellington Formation of Oklahoma, U.S.A.: Implications for paleoclimate and paleogeography of the Midcontinent, J. Sediment. Res., 83, 825–846, https://doi.org/10.2110/jsr.2013.59, 2013.
Glasspool, I. J. and Scott, A. C.: Phanerozoic concentrations of atmospheric oxygen reconstructed from sedimentary charcoal, Nat. Geosci., 3, 627–630, 2010.
Goddéris, Y.: Onset and ending of the late Palaeozoic ice age triggered by tectonically paced rock weathering, Nat. Geosci., 10, 382–388, 2017.
Hamamura, N., Olson, S. H., Ward, D. W., and Inskeep, W. P.: Diversity and functional analysis of bacterial communities associated with natural hydrocarbon seeps in acidic soils at Rainbow Springs, Yellowstone National Park, Appl. Environ. Microbiol., 71, 5943–5950, 2005.
Haq, B. U. and Schutter, S. R.: A chronology of Paleozoic sea-level changes, Science, 322, 64–68, 2008.
Hay, W. W., Migdisov, A., Balukhovsky, A. N., Wold, C. N., Flogel, S., and Soding, E.: Evaporites and the salinity of the ocean during the Phanerozoic: Implications for climate, ocean circulation and Life, Palaeogeogr. Palaeocl., 240, 3–46, https://doi.org/10.1016/j.palaeo.2006.03.044, 2006.
Heavens, N. G., Shields, C. A., and Mahowald, N. M.: Sensitivity of a deep time climate simulation to aerosol prescription, J. Adv. Model. Earth Syst., 4, M11002, https://doi.org/10.1029/2012MS000166, 2012.
Heckel, P. H.: Evidence for global (glacial-eustatic) control over upper Carboniferous (Pennsylvanian) cyclothems in midcontinent North America, Geol. Soc. London Spec. Publ., 55, 35–47, 1990.
Hentz, T. F.: Sequence stratigraphy of the Upper Pennsylvanian Cleveland Formation: A major tight-gas sandstone, western Anadarko Basin, Texas Panhandle, Am. Assoc. Pet. Geol. Bull., 78, 569–595, 1994.
Huang, H., Gao, Y., Jones, M. M., Tao, H., Alan, R., Ibarra, D. E., Huaichun, W., and Wang, C.: Astronomical forcing of Middle Permian terrestrial climate recorded in a large paleolake in northwestern China, Palaeogeogr. Palaeocl., 550, https://doi.org/10.1016/j.palaeo.2020.109735, 2020.
Johnson, K. S.: Anadarko Basin Symposium, Oklahoma Geological Survey, 90, 1–291, 1988.
Juncal, M., Bourquin, S., Beccaletto, L., and Diez, J.: New sedimentological and palynological data from the Permian and Triassic series of the Sancerre-Couy core, Paris Basin, France, Geobios, 51, 517–535, https://doi.org/10.1016/j.geobios.2018.06.007, 2018.
Keranen, K. M. and Weingarten, M.: Induced seismicity, Ann. Rev. Earth Planet. Sci., 46, 149–174, https://doi.org/10.1146/annurev-earth-082517-010054, 2018.
Kiessling, W.: Long-term relationships between ecological stability and biodiversity in Phanerozoic reefs, Nature, 433, 410–413, https://doi.org/10.1038/nature03152, 2005.
Kutzbach., J. E. and Gallimore, R. G.: Pangean climates: megamonsoons of the megacontinent, J. Geophys. Res., 94, 3341–3357, 1989.
Langenbruch, C., Weingarten, M., and Zoback, M. D.: Physics-based forecasting of man-made earthquake hazards in Oklahoma and Kansas, Nat. Commun., 9, 1–10, https://doi.org/10.1038/s41467-018-06167-4, 2018.
Lee, L. A., Reddington, C. L., and Carslaw, K. S.: On the relationship between aerosol model uncertainty and radiative forcing uncertainty, P. Natl. Acad. Sci. USA, 113, 5820–5827, https://doi.org/10.1073/pnas.1507050113, 2016.
Lin, L., Khider, D., Lisiecki, L. E., and Lawrence, C. E.: Probabilistic sequence alignment of stratigraphic records, Paleoceanography, 29, 976–989, https://doi.org/10.1002/2014PA002713, 2014.
Lisiecki, L. E. and Lisiecki, P. A.: Application of dynamic programming to the correlation of paleoclimate records, Paleoceanography, 17, 1049, https://doi.org/10.1029/2001PA000733, 2002.
LIP Commission, http://www.largeigneousprovinces.org/, last access: August 2020.
Liu, X., Xu, T., and Liu, T.: The Chinese loess in Xifeng, II. A study of anisotropy of magnetic susceptibility of loess from Xifeng, Geophys. J. Int., 92, 349–353, https://doi.org/10.1111/j.1365-246X.1988.tb01147.x, 1999.
Luo, Q., Krumholz, L. R., Najar, F. Z., Peacock, A. D., Roe, B. A., White, D. C., and Elshahed, M. S.: Diversity of the microeukaryotic community in sulfide-rich Zodletone Spring (Oklahoma), Appl. Environ. Microbiol., 71, 6175–6184, https://doi.org/10.1128/AEM.71.10.6175-6184.2005, 2005.
Maher, B. A.: Palaeoclimatic records of the loess/paleosol sequences of the Chinese Loess Plateau, Quaternary Sci. Rev., 154, 23–84, 2016.
McArthur, J. M., Howarth, R. J., and Bailey, T. R.: Strontium isotope stratigraphy: LOWESS version 3: best fit to the marine Sr-isotope curve for 0–509 Ma and accompanying look-up table for deriving numerical age, J. Geol., 109, 155–170, 2001.
Menard, G. and Molnar, P.: Collapse of a Hercynian Tibetan Plateau into a late Palaeozoic European Basin and Range province, Nature, 334, 235–237, 1988.
Mercuzot, M.: Reconstitutions paléoenvironnementales et paléoclimatiques en contexte tardi-orogénique des bassins Carbonifères-Permiens du nord du Massif Central, France, PhD thesis, University of Rennes, expected October 2020.
Mercuzot, M., Bourquin, S., Beccaletto, L., Ducassou, C., Rubi, R., and Pellenard, P.: Palaeoenvironmental reconstitutions at the Carboniferous-Permian transition south of the Paris Basin, France: implications on the stratigraphic evolution and basin geometry, Int. J. Earth Sci., accepted, 2020.
Meyers, S. R.: Cyclostratigraphy and the problem of astrochronologic testing, Earth Sci. Rev., 190, 190–223, https://doi.org/10.1016/j.earscirev.2018.11.015, 2019.
Meyers, S. R.: The evaluation of eccentricity-related amplitude modulation and bundling in paleoclimate data: an inverse approach for astrochronologic testing and time scale optimization, Paleoceanography, 30, 1625–1640, https://doi.org/10.1002/2015PA002850, 2015.
Meyers, S. R. and Sageman, B. B.: Quantification of deep-time orbital forcing by average spectral misfit, Am. J. Sci., 307, 773–792, https://doi.org/10.2475/05.2007.01, 2007.
Michel, L. A., Tabor, N. J., Montanez, I. P., Schmitz, M., and Davydov, V. I.: Chronostratigraphy and paleoclimatology of the Lodéve Basin, France: Evidence for a pan-tropical aridification event across the Carboniferous – Permian boundary, Palaeogeogr. Palaeocl., 430, 118–131, https://doi.org/10.1016/j.palaeo.2015.03.020, 2015.
Molnar, P. and England, P.: Late Cenozoic uplift of mountain ranges and global climate change: chicken or egg?, Nature, 346, 29–34, 1990.
Montañez, I. P., Tabor, N. J., Niemeier, D., DiMichele, W. A., Frank, T. D., Fielding, C. R., Isbell, J. L., Birgenheier, L. P., and Rygel, M.: CO2-forced climate and vegetation instability during Late Paleozoic deglaciation, Science, 315, 87–91, 2007.
Montañez, I. P., McElwain, J. C., Poulsen, C. J., White, J. D., DiMichele, W. A., Wilson, J. P., Griggs, G., and Hren, M. T.: Climate, pCO2 and terrestrial carbon cycle linkages during late Palaeozoic glacial-interglacial cycles, Nat. Geosci., 9, 824–828, 2016.
Muttoni, G. and Kent, D. V.: Adrea as promontory of Africa and its conceptual role in the Tethys twist and Pangea B to Pangea A transformation in the Permian, Rivista Italiana di Paleontogia e Stratigrafia, 125, 249–269, 2019.
Nawrocki, J., Polechonska, O., Bogucki, A., and Łanczont, M.: Palaeowind directions recorded in the youngest loess in Poland and western Ukraine as derived from anisotropy of magnetic susceptibility measurements, Boreas, 35, 266–271, https://doi.org/10.1111/j.1502-3885.2006.tb01156.x, 2006.
Nelsen, M. P., DiMichele, W. A., Peters, S. E., and Boyce, C. K.: Delayed fungal evolution did not cause the Paleozoic peak in coal production, P. Natl. Acad. Sci. USA, 113, 2442–2447, 2016.
NRC (National Research Council), Understanding Earth's Deep Past – Lessons for Our Climate Future, National Academies Press, Washington, D.C., 194, 2011.
Pardo, J. D., Small, B. J., Milner, A. R., and Huttenlocker, A. K.: Carboniferous-Permian climate change constrained by early land vertebrate radiations, Nature Ecol. Evol., 3, 200–206, https://doi.org/10.1038/s41559-018-0776-z, 2019.
Parrish, J. T.: Paleoclimate of the supercontinent Pangea, J. Geology, 101, 215–233, 1993.
Parrish, J. T.: Interpreting Pre-Quaternary Climate from the Geologic Record, Columbia University Press, New York, 1998.
Payne, M. E. and Clapham, J. L.: End-Permian mass extinction in the oceans: an ancient analog for the twenty-first century?, Ann. Rev. Earth Planet. Sci., 40, 89–111, https://doi.org/10.1146/annurev-earth-042711-105329, 2012.
Pellenard, P., Gand, G., Schmitz, M, Galtier, J., Broutin, J., and Stéyer, J. S.: High-precision U-Pb zircon ages for explosive volcanism calibrating the NW European continental Autunian stratotype, Gondwana Res., 51, 118–136, 2017.
Pfeifer, L. S., Soreghan, G. S., Pochat, S., Van Den Driessche, J., and Thomson, S. N.: Permian exhumation of the Montagne Noire core complex recorded in the Graissessac-Lodève Basin, France, Basin Research, 30, 1–14, https://doi.org/10.1111/bre.12197, 2016.
Pfeifer, L. S., Soreghan, G. S., Pochat, S., and Van Den Driessche, J.: Paleoclimatic significance of Permian loess in eastern equatorial Pangea: The Lodève Basin (France), Geol. Soc. Am. Bull., https://doi.org/10.1130/B35590.1, 2020a.
Pfeifer, L. S., Hinnov, L. A., Zeeden, C., Rolf, C., Laag, C., and Soreghan, G. S.: Rock magnetic cyclostratigraphy of Permian loess in eastern equatorial Pangea (Salagou Formation, south-central France), Front. Earth Sci., 8, 241, https://doi.org/10.3389/feart.2020.00241, 2020b.
Pochat, S. and Van Den Driessche, J.: Filling sequence in Late Paleozoic continental basins: A chimera of climate change? A new light shed given by the Graissessac–Lodève basin (SE France), Palaeogeogr. Palaeocl., 302, 170–186, 2011.
Praeg, D.: Diachronous Variscan late-orogenic collapse as a response to multiple detachments; a view from the internides in France to the foreland in the Irish Sea, Geol. Soc. Spec. Pub., 223, 89–138, 2004.
Reiners, P. W. and Brandon, M. T.: Using thermochronology to understand orogenic erosion, Ann. Rev. Earth Planet. Sci., 34, 419–466, 2006.
Rondot, A.: An Integrated Geophysical Analysis of Crustal Structure in the Wichita Uplift Region of Southern Oklahoma. M.S. Thesis, Norman, University of Oklahoma, 107 pp., 2009.
Saltzman, M. R. and Thomas, E.: Carbon isotope stratigraphy, in: The Geologic Time Scale 2012, edited by: Gradstein, F. M., Ogg, J. G., Schmitz, M., and Ogg, G., Elsevier, 207–232, 2012.
Sardar Abadi, M., Owens, J. D., Liu, X., Them II, T. R., Cui, X., Heavens, N. G., and Soreghan, G. S.: Atmospheric dust stimulated marine primary productivity during Earth's penultimate icehouse, Geology, 480, 247–251, https://doi.org/10.1130/G46977.1, 2020.
Satterfield, C. L., Lowenstein, T. K., Vreeland, R. H., Rosenzweig, W. D., and Powers, D. W.: New evidence for 250 Ma age of halotolerant bacterium from a Permian salt crystal, Geology, 33, 265–268, 2005.
Scholze, F. and Schneider, J. W.: Improved methodology of “conchostracan” (Crustacea: Branchiopoda) classification for biostratigraphy, Newsletters on Strat., 48, 287–298, 2015.
Schwartz, S. E. and Andreae, M. O.: Uncertainty in climate change caused by aerosols, Science, 272, 1121–1122, 1996.
Sheldon, N. D.: Do red beds indicate paleoclimatic conditions?: A Permian case study, Palaeogeogr. Palaeocl., 228, 305–319, 2005.
Soreghan, G. S., Soreghan, M. J., Poulsen, C. E., Young, R. A., Eble, C., Sweet, D. E., and Davogustto, O.: Anomalous cold in the Pangaean tropics, Geology, 36, 659–662, 2008a.
Soreghan, G. S., Soreghan, M. J., and Hamilton, M. A.: Origin and significance of loess in late Paleozoic western Pangaea: A record of tropical cold?, Palaeogeogr. Palaeocl., 268, 234–259, https://doi.org/10.1016/j.palaeo.2008.03.050, 2008b.
Soreghan, G. S., Keller, G. R., Gilbert, M. C., Chase, C. G., and Sweet, D.: Load-induced subsidence of the Ancestral Rocky Mountains recorded by preservation of Permian landscapes, Geosphere, 8, 654–668, https://doi.org/10.1130/GES00681.S1, 2012.
Soreghan, G. S., Sweet, D. E., and Heavens, N. G.: Upland glaciation in tropical Pangaea: Geologic evidence and implications for Late Paleozoic climate modeling, J. Geology, 122, 137–163, 2014.
Soreghan, G. S., Heavens, N. G., Hinnov, L. A., Aciego, S. M., and Simpson, C.: Reconstructing the dust cycle in deep time: The case of the Late Paleozoic icehouse, in: Earth-Life Transitions – Paleobiology in the Context of Earth System Evolution: The Paleontological Society Papers, edited by: Polly, P. D. and Fox, D. L., 21, 83–120, 2015a.
Soreghan, G. S., Benison, K. C., Foster, T. M., Zambito, J., and Soreghan, M. J.: The paleoclimatic and geochronologic utility of coring red beds and evaporites: a case study from the RKB core (Permian, Kansas, USA), Int. J. Earth Sci., 104, 1–17, https://doi.org/10.1007/s00531-014-1070-1, 2015b.
Soreghan, G. S, Soreghan, M. J., and Heavens, N. G.: Explosive volcanism as a key driver of the Late Paleozoic Ice Age, Geology, 47, 600–604, https://doi.org/10.1130/G46349.1, 2019.
Soreghan, M. J., Soreghan, G. S., and Hamilton, M. A.: Paleowinds inferred from detrital-zircon geochronology of upper Paleozoic loessite, western equatorial Pangea, Geology, 30, 695–698, 2002.
Soreghan, M. J., Heavens, N. G., Soreghan, G. S., Link, P. K., and Hamilton, M. A.: Abrupt and high-magnitude changes in atmospheric circulation recorded in the Permian Maroon Formation, tropical Pangaea, Geol. Soc. Am. Bull., 126, 569–584, https://doi.org/10.1130/B30840.1, 2014.
Soreghan, M. J., Swift, M. M., and Soreghan, G. S.: Provenance of Permian eolian and related strata in the North American Midcontinent: Tectonic and climatic controls on sediment dispersal in western tropical Pangea, in: Tectonics, Sedimentary Basins, and Provenance: A Celebration of the Career of William R. Dickinson, edited by: Ingersoll, R. V., Lawton, T. F., and Graham, S. A., Geol. Soc. Am. Spec. Pap., 540, 661–687, https://doi.org/10.1130/SPE540, 2018.
Steiner, M. B.: The magnetic polarity time scale across the Permian–Triassic boundary, in: Non-Marine Permian Biostratigraphy and Biochronology, edited by: Lucas, S. G., Cassinis, G., and Schneider, J. W., Geol. Soc. London, Spec. Pub., 265, 15–38, 2006.
Sternai, P., Herman, F., Champagnac, J. D., Fox, M., Salcher, B., and Willett, S. D.: Pre-glacial topography of the European Alps, Geology, 40, 1067–1070, https://doi.org/10.1130/G33540.1, 2012.
Sues, H.-D. and Reisz, R. R.: Origins and early evolution of herbivory in tetrapods, Trends Ecol. Evol., 13, 141–145, 1998.
Sun, Y., Lu, H., and An, Z.: Grain size of loess, palaeosol and Red Clay deposits on the Chinese Loess Plateau: Significance for understanding pedogenic alteration and palaeomonsoon evolution, Palaeogeogr. Palaeocl., 241, 129–138, 2006.
Sur, S., Owens, J. D., Soreghan, G. S., Lyons, T. W., Raiswell, R., Heavens, N. G., and Mahowald, N. M.: Extreme eolian delivery of reactive iron to late Paleozoic icehouse seas, Geology, 43, 1099–1102, https://doi.org/10.1130/G37226.1, 2015.
Svensen, H., Planke, S., Polozov, A. G., Schmidbauer, N., Corfu, F., Podladchikov, Y. Y., and Jamtveit, B.: Siberian gas venting and the end-Permian environmental crisis, Earth Planet. Sci. Lett., 277, 490–500, 2009.
Sweet, A. C., Soreghan, G. S., Sweet, D. E., Soreghan, M. J., and Madden, A. S.: Permian dust in Oklahoma: Source and origin for middle Permian (Flowerpot-Blaine) redbeds in western tropical Pangaea, Sediment. Geol., 284–285, 181–196, doi.org/10.1016/j.sedgeo.2012.12.006, 2013.
Tabor, N. J. and Montanez, I. P.: Shifts in late Paleozoic atmospheric circulation over western equatorial Pangea: insights from pedogenic mineral ∂18O compositions, Geology, 30, 1127–1130, 2002.
Tabor, N. J. and Poulsen, C. J.: Palaeoclimate across the Late Pennsylvanian–Early Permian tropical palaeolatitudes: A review of climate indicators, their distribution, and relation to palaeophysiographic climate factors: Palaeogeogr. Palaeocl., 268, 293–310, 2008.
Tabor, N. J., Myers, T. S., Mack, G. H., Looy, C. V., and Renne, P. R.: Quatermaster Formation of north Texas, USA; Part I, Litho- and chemostratigraphy, Abstracts With Programs, Geol. Soc. Am., 43, 383, 2011.
Tabor, N. J. and Myers, T. S.: Paleosols as indicators of paleoenvironment and paleoclimate, Annual Rev. Earth Planet. Sci., 43, 333–361, 2015.
Theiling, B. P., Elrick, M., and Asmerom, Y.: Increased continental weathering flux during orbital-scale sea-level highstands: Evidence from Nd and O isotope trends in middle Pennsylvanian cyclic carbonates, Palaeogeogr. Palaeocl., 342–343, 17–26, https://doi.org/10.1016/j.palaeo.2012.04.017, 2012.
Tian, H., Fan, M., Chamberlain, K. R., Waite, L., and Stern, R. J.: Zircon LA-ICPMS and CA-TIMS U-PB dates of late Paleozoic volcanic tuffs in the Midland Basin, West Texas, Abstracts with Programs, Geol. Soc. Am., 52, 1, https://doi.org/10.1130/abs/2020SC-343618, 2020.
Tomezzoli, R. N., Tickj, H., Rapalini, A. E., Gallo, L. C., Cristallini, E. O., Arzadún, G., and Chemale Jr., F.: Gondwana's Apparent Polar Wander Path during the Permian – new insights from South America, Sci. Reports, 8, 8436, https://doi.org/10.1038/s41598-018-25873-z, 2018.
Twitchett, R. J., Looy, C. V., Morante, R., Visscher, H., and Wignall, P. B.: Rapid and synchronous collapse of marine and terrestrial ecosystems during the end-Permian biotic crisis, Geology, 29, 351–354, 2001.
Valentino, D. W. and Gates, A. E.: Asynchronous extensional collapse of a transpressional orogen: the Alleghanian central Appalachian Piedmont, USA, J. Geodyn., 31, 145–167, 2001.
Walker, T. R.: Formation of red beds in modern and ancient deserts, Geol. Soc. Am. Bull., 78, 353–68, 1967.
Walker, T. R.: Formation of red beds in moist tropical climates: A hypothesis, Geol. Soc. Am. Bull., 85, 633–638, 1974.
Warren, J. K.: Evaporites, A Geological Compendium, Second Edition, Springer International Publishing AG, Switzerland, p. 1813, 2016.
Wignall, P. B. and Twitchett, R. J.: Oceanic anoxia and the end Permian mass extinction, Science, 272, 1155–1158, https://doi.org/10.1126/science.272.5265.1155 1996.
Williams, D. F., Peck, J., Karabanov, E. B., Prokopenko, A. A., Kravchinsky, B., King, J., and Kuzmin, M. I.: Lake Baikal record of continental climate response to orbital insolation during the past 5 million years, Science, 278, 1114–1117, https://doi.org/10.1126/science.278.5340.1114, 1997.
Wilson, L. R.: A Permian fungus spore type from the Flowerpot Formation of Oklahoma, Oklahoma Geology Notes, 22, 91–96, 1962.
Witt, W. J.: Cross section of Oklahoma from SW to NE corners of state: Oklahoma City Geological Society, Oklahoma, 1971.
Wu, H., Fang, Q., Wang, X., Hinnov, L. A., Qi, Y., Shen, S., Yang, T., Li, H., Chen, J., and Zhang, S. A.: ∼ 34 M.y. astronomical time scale for the uppermost Mississippian through Pennsylvanian of the Carboniferous System of the Paleo-Tethyan Realm, Geology, 47, 83–86, https://doi.org/10.1130/G45461.1, 2019.
Wu, H., Zhang, S., Hinnov, L. A., Feng, Q., Jiang, G., Li, H., and Yang, T.: Time-calibration of Milankovitch cycles in the Late Permian, Nat. Commun., 4, 2452, https://doi.org/10.1038/ncomms3452, 2013.
Yang, S. and Ding, Z.: A 249 kyr stack of eight loess grain size records from northern China documenting millennial-scale climate variability, Geochem. Geophy., Geosy., 15, 798–814, https://doi.org/10.1002/2013GC005113, 2013.
Zambito, J. J. and Benison, K. C.: Extremely high temperatures and paleoclimate trends recorded in Permian ephemeral lake halite, Geology, 41, 587–590, https://doi.org/10.1130/G34078.1, 2013.
Zhang, R., Kravchinsky, V. A., Zhu, R., and Yue, L.: Paleomonsoon route reconstruction along a W-E transect in the Chinese Loess Plateau using the anisotropy of magnetic susceptibility: Summer monsoon model, Earth Planet. Sci. Lett., 299, 436–446, https://doi.org/10.1016/j.epsl.2010.09.026, 2010.
Zhu, R., Liu, Q., and Jackson, M. J.: Paleoenvironmental significance of the magnetic fabrics in Chinese loess-paleosol since the last interglacial (< 130 ka), Earth Planet. Sci. Lett., 221, 55–69, https://doi.org/10.1016/S0012-821X(04)00103-7, 2004.
- Abstract
- Introduction
- Compelling science enabled by coring the Permian
- Suitability of archives and indicators
- Site selection
- The need for coring
- Dating potential
- Concluding recommendations
- Data availability
- Team list
- Author contributions
- Competing interests
- Acknowledgements
- Financial support
- Review statement
- References
- Supplement
- Abstract
- Introduction
- Compelling science enabled by coring the Permian
- Suitability of archives and indicators
- Site selection
- The need for coring
- Dating potential
- Concluding recommendations
- Data availability
- Team list
- Author contributions
- Competing interests
- Acknowledgements
- Financial support
- Review statement
- References
- Supplement