the Creative Commons Attribution 4.0 License.
the Creative Commons Attribution 4.0 License.
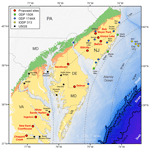
Paleogene Earth perturbations in the US Atlantic Coastal Plain (PEP-US): coring transects of hyperthermals to understand past carbon injections and ecosystem responses
Marci M. Robinson
Kenneth G. Miller
Tali L. Babila
Timothy J. Bralower
James V. Browning
Marlow J. Cramwinckel
Monika Doubrawa
Gavin L. Foster
Megan K. Fung
Sean Kinney
Maria Makarova
Peter P. McLaughlin
Paul N. Pearson
Ursula Röhl
Morgan F. Schaller
Jean M. Self-Trail
Appy Sluijs
Thomas Westerhold
James D. Wright
James C. Zachos
The release of over 4500 Gt (gigatonnes) of carbon at the Paleocene–Eocene boundary provides the closest geological analog to modern anthropogenic CO2 emissions. The cause(s) of and responses to the resulting Paleocene–Eocene Thermal Maximum (PETM) and attendant carbon isotopic excursion (CIE) remain enigmatic and intriguing despite over 30 years of intense study. CIE records from the deep sea are generally thin due to its short duration and slow sedimentation rates, and they are truncated due to corrosive bottom waters dissolving carbonate sediments. In contrast, PETM coastal plain sections along the US mid-Atlantic margin are thick, generally having an expanded record of the CIE. Drilling here presents an opportunity to study the PETM onset to a level of detail that could transform our understanding of this important event. Previous drilling in this region provided important insights, but existing cores are either depleted or contain stratigraphic gaps. New core material is needed for well-resolved marine climate records. To plan new drilling, members of the international scientific community attended a multi-staged, hybrid scientific drilling workshop in 2022 designed to maximize not only scientifically and demographically diverse participation but also to protect participants' health and safety during the global pandemic and to reduce our carbon footprint. The resulting plan identified 10 sites for drill holes that would penetrate the Cretaceous–Paleogene (K–Pg) boundary, targeting the pre-onset excursion (POE), the CIE onset, the rapidly deposited Marlboro Clay that records a very thick CIE body, and other Eocene hyperthermals. The workshop participants developed several primary scientific objectives related to investigating the nature and the cause(s) of the CIE onset as well as the biotic effects of the PETM on the paleoshelf. Additional objectives focus on the evidence for widespread wildfires and changes in the hydrological cycle, shelf morphology, and sea level during the PETM as well as the desire to study both underlying K–Pg sediments and overlying post-Eocene records of extreme hyperthermal climate events. All objectives address our overarching research question: what was the Earth system response to a rapid carbon cycle perturbation?
- Article
(5948 KB) - Full-text XML
- BibTeX
- EndNote
Despite over 30 years of intense study since rapid climatic and biotic change during the Paleocene–Eocene Thermal Maximum (PETM) was first identified (Thomas, 1989; Kennett and Stott, 1991), the cause(s) of and responses to the PETM and attendant stable carbon isotope excursion (CIE) in the global exogenic carbon pool (e.g., Koch et al., 1992; Dickens et al., 1995) remain enigmatic and intriguing. The PETM remains an essential target of climate study, as the release of over 4500 Gt (with Gt = Pg; Gutjahr et al., 2017) of carbon at the Paleocene–Eocene (P–E) boundary provides the closest geological analog to modern anthropogenic CO2 emissions (e.g., Pagani et al., 2006; Zeebe et al., 2016). The PETM was characterized by a global temperature increase of about 5 °C (Kennett and Stott, 1991; Koch et al., 1992; Bralower et al., 1995; Thomas et al., 1999; Zachos et al., 2003; Tripati and Elderfield, 2004; Sluijs et al., 2006; Dunkley Jones et al., 2013; Tierney et al., 2022). The CIE is recorded by a δ13C decrease in marine (∼ 2 ‰–3 ‰ in benthic; ∼ 2.5 ‰–4 ‰ in planktonic foraminifera) and terrestrial (∼ 2.5 ‰–7 ‰) environments, requiring a substantial addition of 13C-depleted carbon into global reservoirs (Kennett and Stott, 1991; Zachos et al., 2007; McInerney and Wing, 2011; Koch et al., 1992; Wing et al., 2005; Bowen et al., 2015). The initiation of the CIE is used to correlate the beginning of the Eocene epoch (∼ 56 Ma).
The PETM CIE can be subdivided into three stages (Röhl et al., 2007; Fig. 1). The first is the CIE onset and a subsequent sharp δ13C decrease, the rapidity of which is still unresolved (e.g., Kennett and Stott, 1991; Bowen et al., 2015; Kirtland Turner and Ridgwell, 2016; Zeebe et al., 2016, Babila et al., 2022; Li et al., 2022). The onset duration estimates range widely (e.g., Cui et al., 2011; Wright and Schaller, 2013), though recent estimates indicate that it occurred in less than 4 kyr (Kirtland Turner, 2018). The second stage, the CIE body (Röhl et al., 2007), is a period of relatively stable low carbon isotope values with astronomical tuning-based estimates on the order of 40 to 100 kyr (Katz et al., 1999; Röhl et al., 2007; Westerhold et al., 2018). The third stage is the gradual exponential recovery period of ∼ 70 to 140 kyr to near pre-CIE δ13C values (Dickens et al., 1997; Katz et al., 1999; Röhl et al., 2007; Westerhold et al., 2018), although several records suggest a faster recovery for at least part of the event (e.g., Bowen and Zachos, 2010). Following the PETM, about 20 smaller negative δ13C excursions (e.g., H1, H2, I1, I2, J, K, L; ∼ 54–53 Ma) occurred in the early Eocene. Several of these have been shown to reflect transient global warming phases (“hyperthermals”; Thomas and Zachos, 2000), such as Eocene Thermal Maximum (ETM) 2 (e.g., Lourens et al., 2005; Sluijs et al., 2009). All the Paleogene CIEs, possibly except for the PETM, were astronomically paced, reflecting continued climate instability in this high CO2 world (e.g., Cramer et al., 2003; Westerhold et al., 2017; Setty et al., 2023).
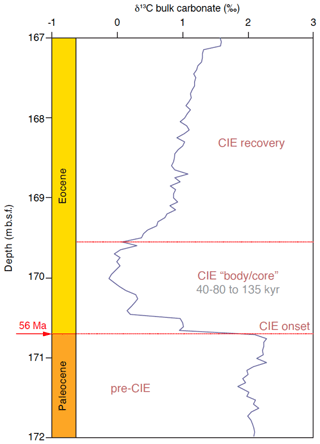
Figure 1Anatomy of the carbon isotope excursion (CIE) using the bulk carbonate δ13C record of Bains et al. (1999) from ODP Site 690, Weddell Sea. This classic deep-sea PETM record illustrates the shape, sediment thickness, and duration of the CIE onset, body/core, and recovery.
Deep-sea sections recording the PETM and other Paleogene hyperthermals are generally thin (< 1 m) due to their geologically short durations and typical deep-sea sedimentation rates of 1–2 cm kyr−1 (Dickens et al., 1995; Westerhold et al., 2018). Records of the CIE onset and body in deep-sea sediments are also generally truncated owing to the corrosive conditions in bottom waters at the onset of the PETM, causing dissolution of calcium carbonate sediments on the seafloor (Zachos et al., 2005; Colosimo et al., 2005; Bralower et al., 2014). In contrast, thick marine sections (> 15 m) recording the CIE occur in the US mid-Atlantic Coastal Plain in New Jersey, Maryland, Delaware, and Virginia. These sections generally have an expanded CIE onset in updip sites, an expanded CIE body in medial sites, and an expanded CIE recovery in the downdip sites due to progradation of sediments (Fig. 2; Podrecca et al., 2021).
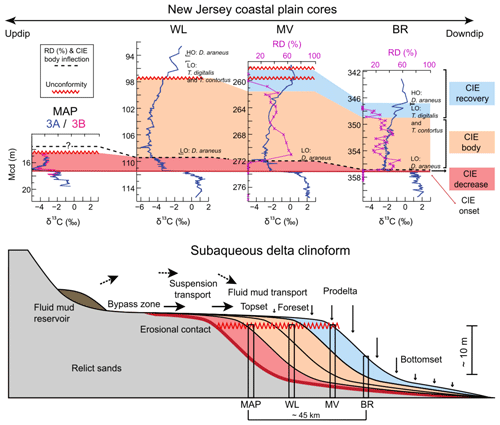
Figure 2(Top) Bulk δ13C records across the four New Jersey coastal plain sites: Medford Auger Project (MAP; 3A in blue and 3B in pink); Wilson Lake B (WL; Wright and Schaller, 2013), Millville (MV; Makarova et al., 2017), and Bass River (BR; Cramer et al., 1999; John et al., 2008); %Rhomboaster–Discoaster (RD; Harris et al., 2010 [MV and BR]; Miller et al., 2017 [WL]); benthic biostratigraphy (Stassen et al., 2015). Records are aligned on the CIE onset (red line), correlated with biostratigraphy, and presented with uniform vertical scale. (Bottom) Idealized subaqueous delta clinoform depositional model depicting fluid mud reservoir source bypassing shallow zones and rapidly building foresets in a prodelta environment. Red saw-toothed line denotes the unconformity capping the top of the Marlboro Clay. Figure from Podrecca et al. (2021).
The prospect of drilling these coastal plain deposits to access data from the expanded PETM sediments led to an International Continental Scientific Drilling Program (ICDP) workshop in 2022, entitled “Coring Paleocene-Eocene Thermal Maximum transects, mid-Atlantic U.S. Coastal Plain: Constraining timing and cause of carbon injection and ecosystem response”. The multi-staged, hybrid scientific drilling workshop was designed to maximize not only scientifically and demographically diverse participation but also to protect participants' health and safety during the global pandemic and to reduce the carbon footprint of this well-attended, international event. This hybrid workshop included (1) a half-day interactive and recorded webinar (22 June, virtually and at Rutgers University, New Brunswick, New Jersey, USA) devoted to discussions related to the science of the PETM and a presentation of potential drill sites; (2) two townhall meetings (24 August, at MARUM, University of Bremen, Germany, during the 12th Climatic and Biotic Events of the Paleogene (CBEP12) conference and 31 August at Grieghallen, Bergen, Norway, during the International Conference in Paleoceanography (ICP14)) where presentations of the preliminary drilling plan and a summary of scientific topics engaged and encouraged additional participation; and (3) a hybrid workshop (17 November, virtually and at Rutgers University, New Brunswick, New Jersey, USA) that finalized the drilling plan and assembled international, discipline-based research teams for post-drilling science.
Across the US mid-Atlantic Coastal Plain, the PETM is associated with the Marlboro Clay, a unique geologic formation of laminated dark gray silty clay. The sediments surrounding the Marlboro Clay, however, vary geographically (Fig. 3). In New Jersey, the lowermost Eocene Marlboro Clay conformably overlies normal sequences of quartzose silty glauconitic sand to glauconitic silt of the Paleocene Vincentown Formation deposited in inner to middle neritic environments (∼ 25–50 m; Harris et al., 2010). The Marlboro Clay is disconformably overlain by either green carbonate clays of the Manasquan Formation or a return to glauconitic sandy silts that are indistinguishable from the Vincentown Formation. In Maryland and Virginia, the Marlboro Clay overlies the greenish-black to greenish-gray very fine to coarse glauconitic sands of the Paleocene Aquia Formation, deposited in inner to middle neritic environments (Gibson and Bybell, 1994). The Aquia Formation–Marlboro Clay contact, coinciding with the Paleocene–Eocene boundary, is usually sharp and highly burrowed, though in New Jersey it is gradational. In Virginia and Maryland, the Marlboro Clay is disconformably overlain by the Nanjemoy Formation, which is lithologically like the Aquia Formation. Previous drilling in Delaware has not identified the presence of the Marlboro Clay, but data presented at the workshops predict a significant thickness near the Maryland border.
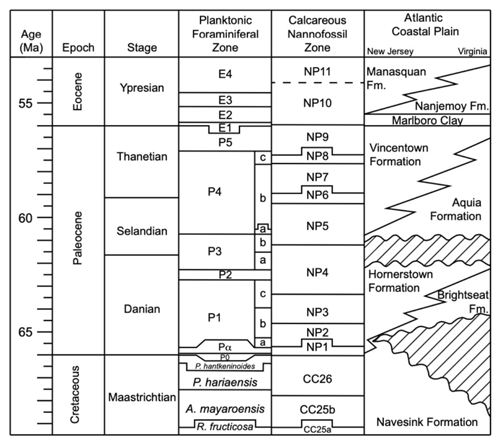
Figure 3Stratigraphic column showing Cretaceous, Paleocene, and Eocene planktonic foraminiferal and calcareous nannofossil zones and geological formation names on the US mid-Atlantic Coastal Plain. Note that same-age sediments in Virginia and New Jersey have different formation names above and below the Marlboro Clay. The Marlboro Clay is closely associated with the PETM across the US mid-Atlantic Coastal Plain.
The Marlboro Clay has a high kaolinite content (Gibson et al., 1993, 2000; Cramer et al., 1999; Lombardi, 2013) that was initially thought to reflect a more vigorous hydrological cycle due to expanded humid tropical conditions during the PETM (Gibson et al., 1993). However, δ18O studies of the clays suggest the kaolinite is recycled from older strata (Kopp et al., 2009; John et al., 2012). The Marlboro Clay also contains magnetic grains like those produced by magnetotactic bacteria on the Amazon shelf and preserved in suboxic zones (Lippert and Zachos, 2007; Kopp et al., 2009), but the dominant source of the magnetic assemblage appears to be non-biotic (Kent et al., 2003, 2017; Wang et al., 2013). The Marlboro Clay, both in composition and flux delivered to the paleoshelf is unusual in the Cenozoic sedimentological history of the US Atlantic Coastal Plain.
The Marlboro Clay was deposited in waters mostly deeper than storm wave base. Exceptions are preserved in updip strata in Virginia and Maryland that record current or storm-wave activity in ripple cross-laminated fine silts that locally exhibit scour and fill channels (Mixon et al., 2000) and the preservation of delta features (Self-Trail et al., 2017). In New Jersey, the Marlboro Clay was deposited in middle to outer neritic paleodepths, primarily on clinoform foresets (Fig. 2; Lombardi, 2013; Stassen et al., 2015; Podrecca et al., 2021). The modern Amazon shelf has been cited as an analog setting for the deposition of the Marlboro Clay (Kopp et al., 2009), exhibiting rapid (2–10 cm yr−1 = 2000–10 000 cm kyr−1) deposition by fluid mud in a subaqueous delta with a clinoform geometry (Nittrouer et al., 1996). The Marlboro Clay contains depositional features consistent with the dominance of fluidized mud depositional processes, including the lack of bioturbation and the rapid burial of vertically oriented pieces of woody debris (Fig. 4). Extremely rapid sediment accumulation (up to 2000 cm yr−1) has been suggested in some intervals (Wright and Schaller, 2013) with the Wilson Lake Hole B sediments indicating that the basal Marlboro Clay represents a very brief interval with anomalously high sedimentation rates (> 12–24 cm yr−1; Miller et al., 2017; Doubrawa et al., 2022). The rate of deposition likely varies across the paleoshelf, and though still not fully quantified, was 1 or 2 orders of magnitude higher than that of concurrent sediments in the deep sea.
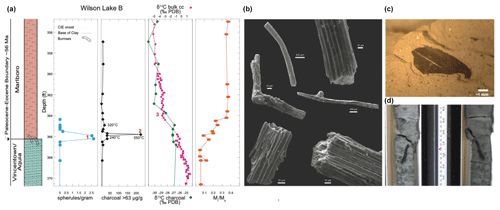
Figure 4(a) Charcoal abundance (no. > 63 µm g−1) and δ13Ccharcoal (Fung et al., 2019) plotted with δ13Cbulk carbonate and impact spherules per gram discovered at Wilson Lake (Schaller et al., 2016). Ratio of saturation remanence to saturation magnetization () at Wilson Lake is interpreted as having been generated by soil pyrogenesis (Kent et al., 2017). (b) SEM images of charcoal fragments recovered from Randalls Farm and Wilson Lake cores from Fung et al. (2019). (c) Photograph of intact leaf fragments from Randalls Farm (Schaller and Fung, 2018). (d) Photograph of split core at Wilson Lake showing upright twig; scale at center.
Previous U.S. Geological Survey (USGS), Ocean Drilling Program (ODP), and Rutgers University coastal plain drilling (Fig. 5) has provided a wealth of basic information and important clues as to the cause(s) of and responses to the PETM. Many USGS cores containing PETM deposits (e.g., Cambridge–Dorchester Airport, Mattawoman Creek–Billingsley Road (MCBR), Randalls Farm, South Dover Bridge, and Wilson Lake Hole A cores) were drilled over the last several decades mainly for the purposes of coastal plain mapping, and their utility for climate studies was only more recently realized. ODP Leg 174AX (e.g., Ancora, Bass River, Millville, Sea Girt cores), similarly, was initially designed to better understand the sequence distribution and architecture of the paleoshelf and slope but also returned PETM sediments that have been key to our present understanding of this interval. The more recent USGS cores (Howards Tract, Knapps Narrows), however, were drilled for climate study as part of the USGS Eocene Hyperthermals Project. Likewise, Wilson Lake Hole B and the Medford Auger Project (Medford core) expressly addressed PETM CIE climate objectives. These more recent efforts represent the beginning of the new phase of PETM climate research on the mid-Atlantic Coastal Plain.
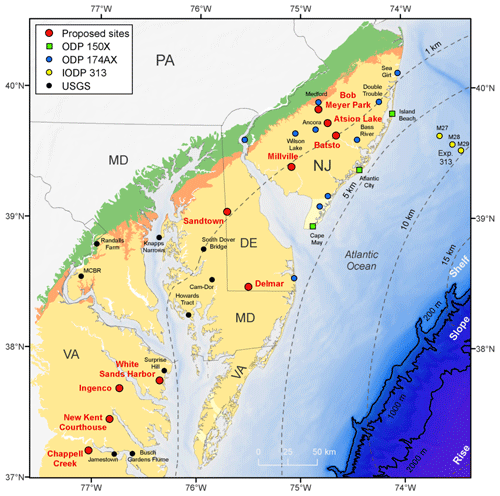
Figure 5Geological map of the US mid-Atlantic Coastal Plain (NJ, New Jersey; DE, Delaware; MD, Maryland; and VA, Virginia) with proposed core sites (red circles) and locations of existing ODP, IODP, and USGS cores. Cam-Dor represents Cambridge–Dorchester Airport; MCBR represents Mattawoman Creek–Billingsley Road. Colors represent outcrops of Cretaceous (green), Paleogene (peach), Neogene (medium yellow), and Quaternary (light yellow) sediments. Blue shades are proportional to water depth. Dashed contours are depth to basement (in km) indicating the direction of dip of sedimentary deposits.
The CIE onset occurs in these cores at the contact between the upper Paleocene Aquia Formation or Vincentown Formation and the lowermost Eocene Marlboro Clay. In the most landward sites in New Jersey and Maryland, the δ13C values across the P–E boundary depict a gradual transition (Self-Trail et al., 2017), but a carbonate dissolution zone in the basal Marlboro Clay found at many deeper sites, possibly due to lysocline shoaling (Bralower et al., 2018), precludes stable isotope records at the contact. However, a 2 ‰ δ13C decrease and ocean acidification event that slightly predates the PETM (the pre-onset excursion or POE; Bowen et al., 2015) records an early pulse of PETM-like conditions in the South Dover Bridge core (Robinson and Spivey, 2019; Babila et al., 2022; Doubrawa et al., 2022), and several smaller CIEs following the PETM are recorded in Maryland (Rush et al., 2023), Virginia, and New Jersey (Fung et al., 2023) sediments. Together, these Eocene hyperthermals provide insight into tipping points in the ecosystem response to rapid change.
One goal of PETM climate research is to place constraints on the timing, magnitude, and tempo of carbon release(s) at the CIE onset (and POE events) and investigate their relationship to each other. However, at Bass River in New Jersey, similar to the deep-ocean records, single-foraminifer stable carbon and oxygen isotope populations are bimodal, showing no intermediate values (Zachos et al., 2007; John et al., 2008) and indicating that foraminifer accumulation rates or foraminifer preservation were insufficient to capture the timescale of 13C-depleted carbon injection. At Mattawoman Creek–Billingsley Road (MCBR), the sedimentation rates are relatively higher than at Bass River, but the foraminifers available for stable isotopic measurements were very small and rare, and many samples were barren (Self-Trail et al., 2017). Foraminifera were more abundant at South Dover Bridge, and δ11B provided a pH proxy, offering a way to trace carbon injection directly (Fig. 6; Babila et al., 2022) but only outside the dissolution zone. The POE was accompanied by a drop in pH and possibly a rise in temperature like during the main CIE (Babila et al., 2022) but was much shorter lived (∼ 2 kyr; Bowen et al., 2015), explaining why deep-sea records fail to capture it. The relatively low deep-sea sedimentation rates and severe chemical erosion at the onset of the main CIE yield insufficient temporal coverage to resolve such a short-lived event. Unfortunately, current late Paleocene stratigraphic age control is poor, and duration estimates between the upper boundary of the POE and main CIE range from 1000 years to 100 kyr (Babila et al., 2022; Doubrawa et al., 2022), limiting our ability to evaluate the connectedness of the multiple carbon injections to the PETM onset.
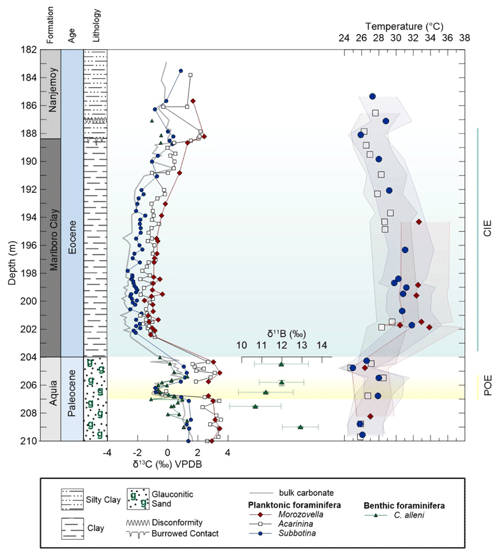
Figure 6South Dover Bridge (SDB) carbon isotope (δ13C) bulk carbonate, planktonic, and benthic foraminifera records. Planktonic foraminifera estimated ocean temperatures and error envelope include uncertainty in seawater composition. Average δ11B of single-specimen benthic foraminifera (Cibicidoides alleni), with 2 SE associated with the mean for each depth and the uncertainty in our internal reference material. Vertical error bars of δ11B represent the full sample depth range of the multiple individual measurements represented by the average δ11B value. Highlighted in yellow is the POE and in blue is the body of the CIE. Note the expanded (> 16 m thick) CIE body compared to < 2 m thickness at ODP Site 690 in Fig. 1 and the dissolution zone (area of no data) at the base of the Marlboro Clay. Figure from Babila et al. (2022).
Another goal is to determine if biotic change, sea surface warming, and the carbon injection associated with the POE were concurrent. Initial micropaleontological and TEX86 studies at Bass River and Wilson Lake (Sluijs et al., 2007) and micropaleontological studies of South Dover Bridge and MCBR (Self-Trail et al., 2017; Robinson and Spivey, 2019; Doubrawa et al., 2022) suggest that biotic change and warming lead the CIE onset by a few millennia. The precursor warming was later reproduced in other regions (e.g., Secord et al., 2010; Frieling et al., 2019). However, TEX86 records from the Medford Auger Project suggest that warming was coincident with or lags the CIE onset (Makarova, 2018; Inglis et al., 2023). Several studies show a gradual, precursor δ13C decrease below the sharp CIE (Miller et al., 2017), with a thickness that increases landward (Fig. 2; Podrecca et al., 2021). Whether temperature rise triggered the carbon release at the POE and main PETM remains an open question.
Irrespective of timing, excess carbon and excessive temperatures affected the ecosystem across the PETM. measurements suggest widespread deoxygenation of thermocline and bottom waters (Zhou et al., 2014; Makarova, 2018), high surface temperatures may have caused the exclusion of eukaryotes in surface waters (Aze et al., 2014; Frieling et al., 2017, 2018; Makarova et al., 2017; Si and Aubry, 2018), and an unusual nannofossil assemblage suggests biotic stress due to photic zone acidification (Kahn and Aubry, 2004; Bralower et al., 2018). Planktonic and benthic organisms showed a significant extinction (benthos) and showed both ecophenotypic (plankton) and ecological (benthos and plankton) responses to the PETM on the paleoshelf, but the cause of the changes is debated.
These ecosystem perturbations are evident on a global scale but can be addressed using records from the US mid-Atlantic Coastal Plain. For example, Makarova et al. (2017) used the transect afforded by Wilson Lake, Ancora, Millville, and Bass River to evaluate two scenarios: (1) a change in the water column structure and (2) a change in habitat or seasonality of the surface dwellers. Si and Aubry (2018) favored a habitat (migration) change, while Makarova (2018) argued for a change in seasonality consistent with the eukaryote exclusion hypothesis. Benthic foraminiferal assemblages suggest dysoxia with an opportunistic CIE fauna (Stassen et al., 2012, 2015; Robinson and Spivey, 2019). data from benthic taxa suggest decreased oxygen concentration but not full anoxia in bottom waters (Makarova, 2018), supporting additional low-oxygen evidence from planktonic/benthic ratios and benthic foraminifera assemblages (Stassen et al., 2012). In addition, reconstructions of paleowater depth based on lithofacies models (Browning et al., 2006), benthic foraminiferal assemblages (Stassen et al., 2015; Robinson and Spivey, 2019), ostracod eye size (Tian et al., 2022), and water depth preferences of planktonic foraminifera (Robinson and Spivey, 2019) indicate a relative sea level rise across the CIE onset that is difficult to explain if ice-free conditions are assumed.
Current available observations of global environmental and ecosystem changes suggest a greater severity both in magnitude and spatial coverage at the PETM when compared to the POE and other Eocene hyperthermals. Two potential causes for a more substantial climate system response proposed are related to external forcing of the carbon cycle. One is related to the release of volcanic carbon inputs, mainly as CO2 associated with the North Atlantic Igneous Province, and preliminary results from drilling on the Norwegian margin strongly supports a link (Planke et al., 2022). The other is extraterrestrial impact. The discovery of in situ impact spherules at the CIE onset at Millville, Wilson Lake, Medford, and deep-sea ODP Site 1051 (Fig. 7; Schaller et al., 2016) offers an intriguing link between extraterrestrial impact and the PETM. The Ar–Ar age of the spherules is indistinguishable from its depositional age at the P–E boundary (Schaller and Fung, 2018; Schaller et al., 2019), indicating that it is not reworked from another impact event. If proximal to the crater, the relatively low abundance of spherules at the CIE onset implies that the volume of CO2 released by this impact was probably not significant. The possibility of a larger impactor cannot be excluded until the ejecta is identified at more far-field P–E sites, though even a small impact could provide a carbon release trigger related to wildfires (Kent et al., 2017; Schaller and Fung, 2018) or earthquakes releasing slope methane (Katz et al., 1999). Fung et al. (2019) documented abundant charcoal in a distinct peak within the CIE onset (Fig. 4) in New Jersey (Wilson Lake) and Virginia (Randalls Farm). Vitrinite reflectance shows that the hottest fires occurred within the peak of charcoal abundance, suggesting they were unusual against the background fire regime of the mid-Atlantic Coastal Plain. These data are tantalizing evidence that, whatever the trigger, wildfires were intense and possibly widespread at the beginning of the PETM climate change. Again, continued research to refine the ages of these sediments would help to understand the cause(s) of the PETM and various environmental perturbations associated with the event onset.
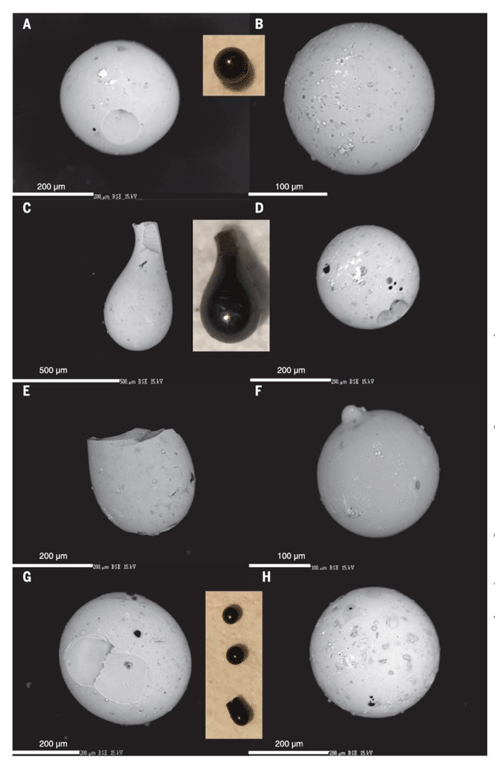
Figure 7Electron backscatter (15 kV) images of representative P–E spherules from IODP Hole 1051B, Wilson Lake B, and Millville cores and the Medford exposure. Selected color micrographs are shown as insets. (a) Microtektite with a surface pit from ODP Hole 1051B. (b) Microkrystite from IODP Hole 1051B. (c) Teardrop-shaped glass spherule from Millville; inset is photomicrograph of same object. (d) Microkrystite with surface pit from Millville. (e) Broken drop-form or dumbbell from Wilson Lake B. (f) Microtektite from Wilson Lake B with a smaller spherule accreted to the side. (g) Microtektite with surface microcrater from the Medford, New Jersey, exposures. Inset shows several other forms found at Medford, which allows us to exclude drilling contamination as a source of the spherules. (h) Typical microkrystite from Wilson Lake B. Figure from Schaller et al. (2016).
In summary, previously drilled cores on the US mid-Atlantic Coastal Plain have provided important clues toward understanding the cause and nature of global change at the PETM, but major open questions still remain. These concern the timing, magnitude, tempo of, and relationship between carbon releases; lead–lag relationships between biotic change and warming and the CIE onset; and the more substantial climate system response of the CIE compared to the POE. These questions can only be fully addressed with additional cores.
The PETM deposits on the US mid-Atlantic Coastal Plain have the potential to provide unparalleled resolution of the shallow marine POE, PETM, and subsequent Eocene hyperthermals. Considering local tectonics and how coastal zone dynamics likely affected PETM deposition, workshop participants designed a drilling campaign of 10 drill holes, with four in New Jersey, two near the Delaware–Maryland border, and four in Virginia, that are intended to capture these events in high resolution (Fig. 5; Table 1). We have assembled extensive well log data for New Jersey, Delaware–Maryland, and Virginia that allow us to confidently predict the presence of the Marlboro Clay (Crider et al., 2022, 2023). Closely spaced well log data are superior to seismic profiling for our purposes because of the thinness (15 m) of the target interval and the fact that it is not imaged on high-resolution 2D and 3D data offshore. We have used existing core holes and well logs from observation wells to construct isopach maps to predict the thickness of the PETM section and to precisely target the proposed core holes in New Jersey and Maryland.
In addition to the PETM and POE, this coring campaign targets Eocene hyperthermals and the K–Pg boundary. Previous coastal plain drilling of ODP Leg 174AX documented the validity of the transect approach (Miller et al., 2017), but existing cores are not adequate for further study. Firstly, the previous cores were drilled for sea-level, hydrostratigraphic, and geologic mapping studies that require less core material for descriptive work than detailed temporal paleoceanographic studies. Bass River and Millville cores, for example, are largely depleted due to intense community sampling of both the working and archive halves, and Wilson Lake Hole B is nearly depleted. Secondly, critical regions from the shallowest paleodepth were either not cored or the sediments were diagenetically altered. Thirdly, unlike ICDP projects and IODP expeditions, no concerted effort was made to coordinate community study or sampling and storing of the PETM intervals.
Therefore, new cores are required to support further PETM research and broaden the potential application of new analyses. New cores will replace existing New Jersey cores that are largely depleted and will supplement the cores currently available in Maryland and Virginia. The southerly of the core sites in Delaware will investigate the record of the PETM between the Maryland-Virginia sites and New Jersey, closer to the core of the Salisbury Embayment. New drilling will complete the depth transects across the paleoshelf (Fig. 5), which is necessary to sample the complex patchwork of sediment and to fully exploit the potential to produce climate records. We will recover the large core volumes that are required for multi-proxy studies and will employ modern high-stratigraphic/temporal-resolution core scanning techniques that are required for astronomical chronology and to resolve environmental changes. Fresh, non-oxidized cores less affected by post-drilling carbonate dissolution will provide the opportunity for additional proxy analyses not possible on existing material (e.g., organic biomarker analysis, inspection of the CIE onset interval for spherules and foraminiferal tests).
4.1 New Jersey
The Marlboro Clay is found in two sediment lobes in New Jersey, indicative of two sediment sources. Our four proposed cores, Millville, Bob Meyer Park in Medford, Atsion Lake, and Batsto, will form a dip transect across the larger, thicker southern lobe from clinoform topset to toes using the predictions of Podrecca et al. (2021; Fig. 2). The existing Millville core is depleted in the onset interval. Therefore, we propose to redrill Millville, a critical location because it provides the most complete CIE onset amongst current mid-Atlantic Coastal Plain core archives and therefore of broad interest to the PETM community. The Medford Auger Project (Podrecca et al., 2021) recovered cores over a 1 km transect near Marlboro Clay outcrops with an expanded CIE onset section. We propose drilling 5.2 km downdip from this transect at Bob Meyer Park in Medford and 19 km downdip at Atsion Lake to recover an expanded CIE onset and to constrain inner shelf conditions. Batsto is 31 km downdip and provides a location between these sites and the existing Bass River site (45 km downdip from outcrop) to record the most expanded CIE body and middle shelf conditions. Bob Meyer Park, Atsion, and Batsto provide an ideal depth transect, with Millville along-strike of Batsto (Fig. 5).
4.2 Delaware–Maryland
The greatest thickness of Paleogene and Neogene sediments lies in the Delmarva Peninsula near Salisbury, Maryland, and these sediments have been largely uncored. Drilling near the Delaware–Maryland border will provide a new wealth of subsurface data. We propose a drill site at Delmar, Delaware, to target the thickest and deepest water deposits sampled in the region. Based on well logs from Federalsburg, Maryland, we predict a thick (> 15 m) PETM section. We also propose an updip site at Sandtown, Delaware, to test the updip extent of the Marlboro Clay. Drilling at the two Delmarva sites, Sandtown and Delmar (Fig. 5), will provide an excellent opportunity for exploratory studies where there is a current knowledge gap and where the nature of the Paleocene–Eocene boundary is not understood.
4.3 Virginia
We used data from numerous wells (i.e., geophysical logs, descriptions of cuttings) to predict the presence and thickness of the Marlboro Clay in Virginia with high confidence. We propose a drill site in White Sands Harbor where Virginia Department of Environmental Quality well data record 67 ft (20 m) of Marlboro Clay. This would represent the thickest Marlboro Clay in the region. Three additional proposed sites at Ingenco, New Kent Courthouse, and Chappell Creek subdivision, with Marlboro Clay thicknesses predicted to be 28 ft (9 m), 30 ft (9 m), and 20 ft (6 m), respectively, will form a deepwater transect that complements existing USGS cores at Surprise Hill, Busch Gardens Flume, and Jamestown (Fig. 5).
Our drilling plan incorporating new material from New Jersey, Delaware, Maryland, and Virginia to complement existing material represents the best opportunity to study the PETM and other hyperthermals in high resolution. The level of detail prescribed in our research approach (see Sect. 6) will transform our understanding of these important events. Our plan, however, is not free from uncertainties and challenges. Differential preservation and patchwork distribution of PETM sediments is expected in coastal plain deposits and can be difficult to predict due to the discontinuous nature of coastal zone sedimentation and erosion. Different intervals of PETM deposition are expected at different locations, depending on the coastal zone dynamics unique to each site. Also, age control is historically challenging in coastal plain sediments due to the generally sparse nature of the microfossils traditionally used for biostratigraphy and stable isotope analyses. Despite these challenges, coastal plain sediments are excellent snapshots of extreme climate states and contain valuable quantitative paleoecological data from peak warm periods. In addition, the preservation of calcium carbonate microfossils is exceptional compared to the deep-sea section due to the dominant clay content limiting dissolution and diagenetic alteration (Pearson et al., 2001).
The biostratigraphic record of Rush et al. (2023; Fig. 8) illustrates the differences in sedimentation rate and preservation of strata at Maryland sites, including the effects of local faulting. Though the US mid-Atlantic coast is a passive continental margin dominated by thermal subsidence and loading, faulting has been mapped in Maryland and Virginia. In some cores, faulting and subsequent erosion is responsible for the absence of some PETM sediments (e.g., in the Knapps Narrows core; Fig. 8). Though there is little evidence of faulting in the New Jersey sections, the US mid-Atlantic margin is affected on the several-million-year scale by relative uplift and subsidence due to changes in mantle dynamic topography (Moucha et al., 2008; Schmelz et al., 2021).
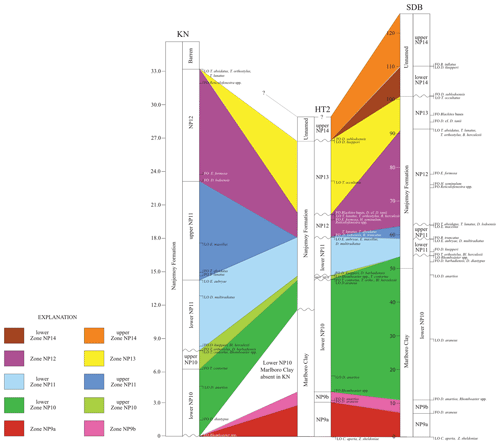
Figure 8Regional calcareous nannofossil biostratigraphy. NP represents nannofossil zones; KN represents Knapps Narrows; HT2 represents Howards Tract 2; SDB represents South Dover Bridge. Thickness of sediments in each core is shown in meters from the base of the PETM. Figure from Rush et al. (2023).
Predicting the nature of sediments to be drilled is always challenging. In the mid-Atlantic Coastal Plain, no seismic profiles are available for a site survey, but seismic profiling is not the best way to detect the ∼ 15 m thick targets at depth. Instead, we have used existing core holes and well logs from observation wells to predict the thickness of the PETM section and to precisely target the proposed core holes in New Jersey and Virginia. On the other hand, while well data control is limited near the proposed Delaware–Maryland sites, drilling here will provide an excellent opportunity for exploratory studies. These sites admittedly pose the greatest risk of missing our target strata, but they also offer the most potential to gain new information.
Finally, age control can be difficult in coastal plain sediments. One fundamental goal is to establish the stratigraphic framework of the coastal plain sediments and to correlate them between and among sites. Core descriptions and age control will provide the basis for all further analyses. Bulk δ13C records, zircon and apatite geochronology, and X-ray fluorescence (XRF) scans will provide a means of evaluating equivalency on the transects. The impact spherules can serve as an isochronous marker horizon to compare between sites. Coupled with careful sedimentologic and stratigraphic documentation, this will enable analyses of the nature, timing, and duration of the PETM onset, our primary science focus.
The PETM, POE, and Eocene hyperthermals provide an excellent template for evaluation of the Earth system response to rapid carbon release at varying degrees of global warming under different background climate states during the Paleogene. The targeted sedimentary strata will allow for evaluation of leads vs. lags in the climate system across a regional scale transect of the mid-latitudes during these differing intervals of rapid global warming. Given the very high temporal resolution (sub-millennial scale), the material to be recovered by the drilling campaign is uniquely situated to answer several questions with direct relevance to anthropogenic climate change. Our overarching question asks: what was the Earth system response to rapid carbon cycle perturbations?
6.1 What was the nature of the CIE onset?
The Marlboro Clay and the transition to it from the underlying units represents a period of unequivocally rapid deposition that provides an expanded record of the carbon release(s) at the PETM onset. Still unknown is the nature of the heterogeneity in the CIE magnitude across shelf deposits, and estimates of the CIE onset duration are wide-ranging. Sampling the transition zone and Marlboro Clay will allow for testing of contrasting hypotheses: one proposing that the main CIE carbon release was virtually instantaneous (Wright and Schaller, 2013) and the other suggesting that it was protracted over ∼ 4 kyr (Kirtland Turner, 2018; Pearson and Nicholas, 2013; Pearson and Thomas, 2015; Zeebe et al., 2016; Li et al., 2022). New cores will provide material needed to attain exceptional resolution of the CIE onset and will be evaluated to determine the speed of the onset and also any lead–lag relationships of carbon injection, temperature rise, biotic and sedimentation change, and extraterrestrial impacts.
Although the POE has been observed in a number of locations, questions remain about the full magnitude of the carbon isotope excursion, whether the carbon released was regional or global, and if it was one or multiple carbon injections that preceded the main CIE or merely a consequence of bioturbation or some other artifact. By measuring stable isotopes in foraminifera, including single-specimen analyses, from several sections on transects across the shelf, we will constrain the true size of the POE, regional expression, and stratigraphic coherence relative to the main CIE. This relative role of forcings and feedbacks is critical in understanding the rate and mechanism(s) of carbon released at the PETM. In addition, we will investigate the relationship between the POE, the CIE recorded at the PETM onset, and the Eocene hyperthermals. We will target sediments likely to contain the POE to determine its relationship to the CIE onset and if it is global and isochronous with previous POE observations from terrestrial records, as well as whether it was a unique event or if there is evidence for multiple carbon injections being responsible for warming at the PETM.
We also propose to determine the origin of the low carbonate and generally carbonate fossil-free interval during the CIE onset (Fig. 6) and how that affects its characterization. We will do this by testing the hypotheses that the carbonate free interval is due to (1) ocean acidification and/or carbonate compensation depth shoaling into the photic zone, (2) dilution by massive input of terrigenous silty clays, or (3) the absence of eukaryotic phytoplankton due to extreme temperatures and ecological exclusion. Our transect approach sampling different paleodepths (nearshore, middle, and outer paleoshelf) in three settings (New Jersey, Delaware–Maryland, and Virginia) will yield constraints at different sites, quantifying dissolution, dilution, and abundance of eukaryotic phytoplankton (foraminifera, nannofossils, and dinoflagellates).
6.2 What was the cause of the CIE onset?
All Eocene hyperthermals initiated during maxima in orbital eccentricity, but whether or not the PETM did is still under debate (Cramer et al., 2003; Zachos et al., 2010; Zeebe and Lourens, 2019; Li et al., 2022; Piedrahita et al., 2022). An orbital trigger superimposed on the long-term late Paleocene-early Eocene warming trend might also be consistent with the magnitude difference between the PETM and subsequent smaller hyperthermals (e.g., Lunt et al., 2011), although the PETM remains exceptionally large. Mathematical analyses on deep-ocean foraminifer δ13C and δ18O records suggest a loss of Earth system resilience in the run-up to the PETM, ETM2, and ETM3, suggesting a carbon source that can be perturbed with climate and carbon cycle variability at the Earth's surface (Setty et al., 2023). But which mechanisms were in place and what was the potential role of North Atlantic Igneous Province volcanisms and even impacts? If the CIE onset occurred during an eccentricity maximum, this may have enhanced the sensitivity of the system to greenhouse gas forcing. The POE and other (biotic) precursors to the CIE may be indicators of an unstable climate system, characterized by a loss of resilience, with a subsequent larger response (the PETM) to a lesser forcing (Setty et al., 2023; Armstrong McKay and Lenton, 2018). We will test the hypothesis that an impact triggered carbon release that, in concert with a preconditioned system and possible astronomical forcing, resulted in a large carbon cycle event. Widespread wildfires associated with an impact would have been synchronous throughout the region. We will test the relationships among carbon release, impact, and wildfires by evaluating the relationships among δ13C, charcoal occurrences, and microtektites.
6.3 What were the biotic effects of the CIEs on the paleoshelf?
We will quantify the severity of the biotic effects of the PETM, POE, and Eocene hyperthermals on the paleoshelf and attempt to recognize ecological tipping points. We will test the hypotheses that there was widespread deoxygenation of the thermocline and bottom waters, an absence of eukaryotes in surface waters due to excessive temperature, and photic zone acidification and biotic stress. The debate surrounding these hypotheses highlights a need for greater community involvement through common study of a series of cores containing nannofossils, planktonic and benthic foraminifera, and dinoflagellate cysts. The proposed transects will allow for a comparison between the degree of biotic effects latitudinally and across a range of water depths. The Paleocene and Eocene shelf sections will provide a detailed and high-resolution baseline for paleoenvironmental conditions, placing POE, PETM, and Eocene hyperthermal excursions into geological context. Establishing the background variability of paleoshelf environments (providing a baseline of the background) is essential to establishing thresholds that cause a major biotic response. Though orbital and other forcings cause hyperthermals, ecological tipping points affect the biotic response, with smaller events causing little disruption and major events like the PETM and ETM2 causing major disruptions.
6.4 How was the hydrological cycle affected by PETM and other hyperthermal perturbations?
We will test whether the intensification of the hydrologic cycle, particularly the increase in seasonality (Rush et al., 2021), drove increased sedimentation or if increased sediment input was due to impact and wildfires in the hinterland. Our drilling will improve estimates of the volume of terrestrial sediments deposited on the shelf, and analyses of hydrogen isotopes of leaf wax n-alkanes will help to reconstruct hydrological cycling. These estimates will be essential for validating hydrologic cycle models of the PETM and testing depositional models. Terrestrial PETM investigations will also benefit from drilling in the coastal plain, as this shallow marine environment provides direct linkages between marine and terrestrial responses to the K–Pg, PETM, and Eocene hyperthermals. Vimpere et al. (2023) provided evidence for continental-scale paleodrainage changes in response to the PETM. Their results from the Gulf of Mexico suggest a strong sedimentary response to changes in the hydrological cycle and also the tectonic regimes that propagated over North America. Mineralogical and isotopic analyses sampling different Marlboro Clay sediment lobes (depocenters) will constrain the provenance of the Marlboro Clay. Did the Potomac, Susquehanna, and Delaware paleorivers tap different Appalachian source regions? We will also consider tectonic-focused studies that examine the potential for the rejuvenation of relief in the Appalachians during the Neogene, as recorded by proxies for chemical weathering in coastal plain sediments.
6.5 How much did relative sea level rise across the PETM?
Multiple lines of evidence argue for a large relative sea level rise in this region across the PETM (Browning et al., 2006; Stassen et al., 2015; Robinson and Spivey, 2019; Tian et al., 2022). We assume that the fall line, the western boundary of the Atlantic Coastal Plain, approximates the shoreline during the late Paleocene and early Eocene, as this is the extent of marine deposits, but the amount of relative sea level rise in the southern (e.g., Virginia) and northern (e.g., New Jersey) regions is not well constrained nor is the relationship between regional and global mean sea level rise (Sluijs et al., 2008). We will use two-dimensional backstripping to reconstruct the paleoslope and place water-depth estimates into a geological context using both inverse techniques (Steckler et al., 1999) and the forward model of Schmelz et al. (2024). We will test our forward model with reconstructions of paleowater depth based on lithofacies models, benthic foraminiferal assemblages, ostracod eye size, and water depth preferences of planktonic foraminifera. Our paleoslope modeling, validating, and refining will be critical in evaluating the response across the paleoshelf to the major warming events of the POE, PETM, and Eocene hyperthermals. Our modeling will also allow us to test the role of changes in global mean sea level versus mantle dynamic topography in controlling deposition during the largely ice-free world of the Paleocene to early Eocene and subsequent development of middle Eocene to Oligocene ice sheets (Miller et al., 2020; Schmelz et al., 2021).
6.6 Can we detect K–Pg seiche influence or warming due to Deccan volcanism?
Because most of the lower Paleocene is condensed or missing, our PETM drilling places us within 30 m of potentially complete K–Pg marine records. By extending our drilling by 30 m, we will be able to test the hypothesis that K–Pg seiche/tsunami wave influence was restricted to paleodepths of ∼ 60 m or less and that deeper sites contain in situ deposits with ballistic ejecta. Previous studies have established Bass River, New Jersey, as a world-class location to sample ballistic ejecta from the K–Pg boundary (Olsson et al., 1997, 2002; Esmeray-Senlet et al., 2015, 2017), and we plan to sample through the K–Pg boundary in our core holes. The mid-Atlantic Coastal Plain offers sampling of intermediate field response (e.g., Schulte et al., 2010) to the K–Pg impact with evidence of seiche/swash back deposits. The most downdip site (Bass River) provides a record of deposition of undisturbed spherule ballistic ejecta immediately following impact (Olsson et al., 1997). Intermediate sites (e.g., Ancora) contain reworked spherules and evidence of seiche/swash back deposits, whereas the most updip sites are either diastemic or have iridium at lower concentration over intervals of tens of centimeters due to bioturbation (Esmeray-Senlet et al., 2015, 2017). The proposed Atsion site is likely to recover seiche/swash back deposits, and the proposed Batsto site should provide a continuous record like Bass River. These cores should recover evidence of the latest Maastrichtian warming event associated with Deccan volcanism ∼ 300 kyr before the K–Pg boundary (e.g., Barnet et al., 2018); this warming was recorded and calibrated to magnetostratigraphy at Bass River (Olsson et al., 2002). A high-resolution record from the uppermost Cretaceous could inform whether this event caused significant ecosystem changes prior to the asteroid impact. The K–Pg will not be sampled in Maryland and Virginia sites where the upper Cretaceous and lower Danian is truncated, and we suspect that it is similarly truncated in Delaware.
6.7 What can we learn from post-Eocene climate records?
Post-Eocene sediments will be of value to ongoing studies focused on the Late Pliocene and Middle Miocene, intervals of past global warmth that are similar to our modern climate in terms of the rate of change and/or the magnitude of atmospheric CO2 concentration and average global temperature. The mid-Piacenzian warm period (∼ 3.3 to 3.0 Ma) during the Late Pliocene represents a final warm pulse before Earth's climate deteriorated into Pleistocene glacial–interglacial cycles, and the Miocene Climatic Optimum (∼ 17–15 Ma) was a warm period atop an already warm climate, similar to our current warming. Climate transitions into the mid-Piacenzian warm period (rapid warming at ∼ 3.3–3.2 Ma) and out of the Miocene Climatic Optimum (slow cooling during the Middle Miocene Climate Transition; ∼ 15–13 Ma) are also valuable targets as they represent rapid changes in global temperature, sea level, ice volume, and oceanic circulation. Previous analyses from the mid-Atlantic Coastal Plain indicate that various foraminifer and biomarker-based proxies provide high-quality reconstructions for these time periods (de Bar et al., 2019; Dowsett et al., 2021; Robinson et al., 2022).
The Paleocene–Eocene Thermal Maximum (PETM) is an essential target of climate study because it is the closest geological analog to modern anthropogenic CO2 emissions. Even after decades of study, however, the cause(s) of and responses to the PETM remain enigmatic and intriguing. Because the rate of deposition of the PETM sediments on the US mid-Atlantic shelf was at least an order of magnitude higher than that of concurrent sediments in the deep sea, coastal plain sediments have the potential to resolve centennial and up to decadal climatic variability and to provide exceptional (sub-millennial) temporal resolution. The discontinuous nature of coastal sedimentation, however, requires numerous sites to constrain events like the POE, PETM, and Eocene hyperthermals.
Workshop participants identified 10 sites for PETM drill holes that target the POE, the CIE onset, the rapidly deposited Marlboro Clay, and other Eocene hyperthermals. To reduce the uncertainty in recovering our target interval, we used data from existing core holes and extensive well logs to construct isopach maps and precisely target thick PETM sections in eight of the proposed core holes. Drilling two cores where little data are available will provide an excellent opportunity to gain pioneering new information. In our post-drilling research, we will not only address the PETM as the most prominent carbon isotope excursion and carbon release of the Cenozoic, but we will also address multiple examples of rapid warming in the latest Paleocene to early Eocene. Though the PETM is arguably the best analog to our rapidly warming climate, the POE and early Eocene hyperthermals provide additional points of comparison for other anthropogenic carbon emission scenarios. Each provides valuable insights into different Earth system responses and feedbacks.
Well log data used to predict the presence of the Marlboro Clay in Maryland and Virginia can be found in a U.S. Geological Survey Data Release at https://doi.org/10.5066/P9AHP9BC (Crider et al., 2022). ODP Leg 150X and ODP Leg 174X data used to predict Marlboro Clay thickness in New Jersey are available through the ODP website. Additional well log data for New Jersey and Delaware are unpublished and reside at Rutgers University and with the New Jersey and Delaware state geological surveys.
MMR and KGM prepared the workshop report with contributions from all co-authors.
The contact author has declared that none of the authors has any competing interests.
Publisher’s note: Copernicus Publications remains neutral with regard to jurisdictional claims made in the text, published maps, institutional affiliations, or any other geographical representation in this paper. While Copernicus Publications makes every effort to include appropriate place names, the final responsibility lies with the authors.
We acknowledge ICDP for funding the workshop entitled “Coring Paleocene–Eocene Thermal Maximum transects, mid-Atlantic U.S. Coastal Plain: Constraining timing and cause of carbon injection and ecosystem response”. We thank Rutgers University and the organizers of the 12th Climatic and Biotic Events of the Paleogene (CBEP12) conference at MARUM, University of Bremen, and the International Conference in Paleoceanography (ICP14) at Grieghallen, Bergen, for hosting the in-person components of our workshop. Finally, we thank Brian Huber and Christian Zeeden for providing insightful reviews that improved the quality of the paper.
This paper was edited by Ulrich Harms and reviewed by Brian Huber and Christian Zeeden.
Armstrong McKay, D. I. and Lenton, T. M.: Reduced carbon cycle resilience across the Palaeocene–Eocene Thermal Maximum, Clim. Past, 14, 1515–1527, https://doi.org/10.5194/cp-14-1515-2018, 2018.
Aze, T., Pearson, P. N., Dickson, A. J., Badger, M. P. S., Bown, P. R., Pancost, R. D., Gibbs, S. J., Huber, B. T., Leng, M. J., Coe, A. L., Cohen, A. S., and Foster, G. L.: Extreme warming of tropical waters during the Paleocene–Eocene Thermal Maximum, Geology, 42, 739–742, https://doi.org/10.1130/G35637.1, 2014.
Babila, T. L., Penman, D. E., Standish, C. D., Doubrawa, M., Bralower, T. J., Robinson, M. M., Self-Trail, J. M., Speijer, R. P., Stassen, P., Foster, G. L., and Zachos, J. C.: Surface ocean warming and acidification driven by rapid carbon release precedes Paleocene-Eocene Thermal Maximum, Science Advances, 8, eabg1025, https://doi.org/10.1126/sciadv.abg1025, 2022.
Bains, S., Corfield, R. M., and Norris, R. D.: Mechanisms of climate warming at the end of the Paleocene, Science, 285, 724–727, https://doi.org/10.1126/science.285.5428.724, 1999.
Barnet, J. S. K., Littler, K, Droon, D., Leng, M. J., Westerhold, T., Rohl, U., and Zachos, J. C.: A new high-resolution chronology for the late Maastrichtian warming event: Establishing robust temporal links with the onset of Deccan volcanism, Geology, 46, 147–150, https://doi.org/10.1130/G39771.1, 2018.
Bowen, G. J. and Zachos, J. C.: Rapid carbon sequestration at the termination of the Palaeocene-Eocene Thermal Maximum, Nat. Geosci., 3, 866–869, 2010.
Bowen, G. J., Maibauer, B. J., Kraus, M. J., Röhl, U., Westerhold, T., Steimke, A., Gingerich, P.D., Wing, S. L., and Clyde, W. C.: Two massive, rapid releases of carbon during the onset of the Palaeocene–Eocene thermal maximum, Nat. Geosci., 8, 44–47, https://doi.org/10.1038/ngeo2316, 2015.
Bralower, T. J., Zachos, J. C., Thomas, E., Parrow, M., Paull, C. K., Kelly, D. C., Premoli Silva, I., Sliter, W. V., and Lohmann, K. C.: Late Paleocene to Eocene paleoceanography of the equatorial Pacific Ocean: stable isotopes recorded at Ocean Drilling Program Site 865, Allison Guyot, Paleoceanography, 10, 841–865, https://doi.org/10.1029/95PA01143, 1995.
Bralower, T. J., Kelly, D. C., Gibbs, S., Farley, K., Eccles, L., Lindemann, T. L., and Smith, G. J.: Impact of dissolution on the sedimentary record of the Paleocene–Eocene thermal maximum, Earth Planet. Sc. Lett., 401, 70–82, https://doi.org/10.1016/j.epsl.2014.05.055, 2014.
Bralower, T. J., Kump, L. R., Self-Trail, J. M., Robinson, M. M., Lyons, S., Babila, T., Ballaron. E., Freeman, K. H., Hajek, E., Rush, W., and Zachos, J. C.: Evidence for shelf acidification during the onset of the Paleocene-Eocene Thermal Maximum, Paleoceanography and Paleoclimatology, 33, 1408–1426, https://doi.org/10.1029/2018PA003382, 2018.
Browning, J. V., Miller, K. G., McLaughlin, P. P., Kominz, M. A., Sugarman, P. J., Monteverde, D., Feigenson, M. D., and Hernàndez, J. C.: Quantification of the effects of eustasy, subsidence, and sediment supply on Miocene sequences, Mid-Atlantic margin of the United States, Geol. Soc. Am. Bull., 118, 567–588, https://doi.org/10.1130/B25551.1, 2006.
Colosimo, A. B, Bralower, T. J., and Zachos, J. C.: Evidence for lysocline shoaling at the Paleocene/Eocene Thermal Maximum on Shatsky Rise, Northwest Pacific, http://www-odp.tamu.edu/publications/198_SR/112/112.htm (last access: 31 January 2024), 2005.
Cramer, B. S., Aubry, M.-P., Miller, K. G., Olsson, R. K., Wright, J. D., and Kent, D. V.: An exceptional chronologic, isotopic, and clay mineralogic record at the latest Paleocene thermal maximum, Bass River, NJ, ODP 174AX, B. Soc. Geol. Fr., 170, 883–897, 1999.
Cramer, B. S., Wright, J. D., Kent, D. V., and Aubry, M.-P.: Orbital climate forcing of δ13C excursions in the late Paleocene–early Eocene (chrons C24n–C25n), Paleoceanography, 18, 1097, https://doi.org/10.1029/2003PA000909, 2003.
Crider, E. A., Self-Trail, J. M., Parker, M., Seefelt, E. L., Staley, A., Beach, T., Bruce, T. S., and Quinn, H.: Database for the isopach map of the Brightseat Formation and structure contour map of the Cretaceous-Paleogene boundary, in Maryland and Virginia, U.S. Geological Survey Data Release [data set], https://doi.org/10.5066/P9AHP9BC, 2022.
Crider, E. A., Self-Trail, J. M., Parker, M., Gardner, K. F., Beach, T., Bruce, T. S., Staley, A., and Quinn, H.: Isopach contour map of the upper Paleocene Aquia Formation and structure contour map of the Paleocene-Eocene boundary in the Salisbury Embayment of Maryland and Virginia, GSA SE/NE Sectional Meeting, Reston, VA, 17–19 March 2023, https://doi.org/10.1130/abs/2023SE-385653, 2023.
Cui, Y., Kump, L. R., Ridgwell, A. J., Charles, A. J., Junium, C. K., Diefendorf, A. F., Freeman, K. H., Urban, N. M., and Harding, I. C.: Slow release of fossil carbon during the Palaeocene–Eocene Thermal Maximum, Nat. Geosci., 4, 481–485, https://doi.org/10.1038/NGEO1179, 2011.
de Bar, M. W., de Nooijer, L. J., Schouten, S., Ziegler, M., Sluijs, A., and Reichart, G.-J.: Comparing Seawater Temperature Proxy Records for the Past 90 Myrs from the Shallow Shelf Record Bass River, New Jersey, Paleoceanography and Paleoclimatology, 34, 455–475, https://doi.org/10.1029/2018PA003453, 2019.
Dickens, G. R., O'Neil, J. R., Rea, D. K., and Owen, R. M.: Dissociation of oceanic methane hydrate as a cause of the carbon isotope excursion at the end of the Paleocene, Paleoceanography, 10, 965–971, https://doi.org/10.1029/95PA02087, 1995.
Dickens, G. R., Castillo, M. M., and Walker J. C. G.: A blast of gas in the latest Paleocene: Simulating first-order effects of massive dissociation of oceanic methane hydrate, Geology, 25, 259–262, https://doi.org/10.1130/0091-7613(1997)025<0259:ABOGIT>2.3.CO;2, 1997.
Doubrawa, M., Stassen, P., Robinson, M. M., Babila, T. L., Zachos, J. C., and Speijer, R. P.: Shelf ecosystems along the U.S. Atlantic Coastal Plain prior to and during the Paleocene-Eocene Thermal Maximum: Insights into the stratigraphic architecture, Paleoceanography and Paleoclimatology, 37, e2022PA004475, https://doi.org/10.1029/2022PA004475, 2022.
Dowsett, H. J., Robinson, M. M., Foley, K. M., and Herbert, T. D.: The Yorktown Formation: Improved Stratigraphy, Chronology, and Paleoclimate Interpretations from the U.S. Mid-Atlantic Coastal Plain, Geosciences, 11, 486, https://doi.org/10.3390/geosciences11120486, 2021.
Dunkley Jones, T., Lunt, D. J., Schmidt, D. N., Ridgwell, A., Sluijs, A., Valdes, P. J., and Maslin, M.: Climate model and proxy data constraints on ocean warming across the Paleocene-Eocene Thermal Maximum, Earth-Sci. Rev., 125, 123–145, https://doi.org/10.1016/j.earscirev.2013.07.004, 2013.
Esmeray-Senlet, S., Wright, J. D., Olsson, R. K., Miller, K. G., Browning, J. V., and Quan, T. M.: Evidence for reduced export productivity following the Cretaceous/Paleogene mass extinction, Paleoceanography, 30, 1–21, https://doi.org/10.1002/2014PA002724, 2015.
Esmeray-Senlet, S., Miller, K. G., Sherrell, R. M., Senlet, T., and Vellekoop, J., Iridium profiles and delivery across the Cretaceous/Paleogene boundary, Earth Planet. Sc. Lett., 27, 117–126, https://doi.org/10.1016/j.epsl.2016.10.010, 2017.
Frieling, J., Gebhardt, H., Huber, M., Adekeye, O. A., Akande, S. O., Reichart, G.-J., Middelburg, J. J., Schouten, S., and Sluijs, A.: Extreme warmth and heat-stressed plankton in the tropics during the Paleocene-Eocene Thermal Maximum, Science Advances, 3, e1600891, https://doi.org/10.1126/sciadv.1600891, 2017.
Frieling, J., Reichart, G.-J., Middelburg, J. J., Röhl, U., Westerhold, T., Bohaty, S. M., and Sluijs, A.: Tropical Atlantic climate and ecosystem regime shifts during the Paleocene–Eocene Thermal Maximum, Clim. Past, 14, 39–55, https://doi.org/10.5194/cp-14-39-2018, 2018.
Frieling, J., Peterse, F., Lunt, D. J., Bohaty, S. M., Sinninghe Damsté, J. S., Reichart, G.-J., and Sluijs, A.: Widespread warming before and elevated barium burial during the Paleocene-Eocene Thermal Maximum: Evidence for methane hydrate release?, Paleoceanography and Paleoclimatology, 34, 546–566, https://doi.org/10.1029/2018pa003425, 2019.
Fung, M. K., Katz, M. E., Miller, K. G., Browning, J. V., and Rosenthal Y.: Sequence stratigraphy, micropaleontology, and foraminiferal geochemistry, Bass River, New Jersey paleoshelf, USA: Implications for Eocene ice-volume changes, Geosphere, 15, 502–532, https://doi.org/10.1130/GES01652.1, 2019.
Fung, M. K., Katz, M. E., Miller, K. G., Browning, J. V., and Schaller, M. F.: Exploring Early Eocene hyperthermals on the New Jersey paleoshelf (ODP 174AX), J. Foramin. Res., 53, 378–396, https://doi.org/10.2113/gsjfr.53.4.378, 2023.
Gibson, T. G. and Bybell, L. M.: Sedimentary patterns across the Paleocene-Eocene boundary in the Atlantic and Gulf Coastal Plains of the United States, Bulletin – Societe Belge de Geologie, 103, 237–265, 1994.
Gibson, T. G., Bybell, L. M., and Owens, J. P.: Latest Paleocene lithologic and biotic events in neritic deposits from south-western New Jersey, Paleoceanography, 8, 495–514, 1993.
Gibson, T. G., Bybell, L. M., and Mason, D. B.: Stratigraphic and climatic implications of clay mineral changes around the Paleocene/Eocene boundary of the northeastern US margin, Sediment. Geol., 134, 65–92, 2000.
Gutjahr, M., Ridgwell, A., Sexton, P. F., Anagnostou, E., Pearson, P. N., Pälike, H., Norris, R. D., Thomas, E., and Foster, G. L.: Very large release of mostly volcanic carbon during the Palaeocene-Eocene Thermal Maximum, Nature, 548, 573–577, https://doi.org/10.1038/nature23646, 2017.
Harris, A. D., Miller, K. G., Browning, J. V., Sugarman, P. J., Olsson, R. K., Cramer, B. S., and Wright, J. D.: Integrated stratigraphic studies of Paleocene-lowermost Eocene sequences, New Jersey Coastal Plain: Evidence for glacioeustatic control, Paleoceanography, 25, PA3211, https://doi.org/10.1029/2009PA001800, 2010.
Inglis, G. N., Martinez-Sosa, P., Tierney, J. E., Witkowski, C. R., Lyons, S., Baczynski, A. A., and Freeman, K. H.: Impact of organic carbon reworking upon GDGT temperature proxies during the Paleocene-Eocene Thermal Maximum, Org. Geochem., 183, 104644, https://doi.org/10.1016/j.orggeochem.2023.104644, 2023.
John, C. M., Bohaty, S. M., Zachos, J. C., Sluijs, A., Gibbs, S., Brinkhuis, H., and Bralower, T. J.: North American continental margin records of the Paleocene-Eocene thermal maximum: Implications for global carbon and hydrological cycling, Paleoceanography, 23, 1–20, https://doi.org/10.1029/2007PA001465, 2008.
John, C. M., Banerjee, N. R., Longstaffe, F. J., Sica, C., Law, K. R., and Zachos, J. C.: Clay assemblage and oxygen isotopic constraints on the weathering response to the Paleocene- Eocene thermal maximum, east coast of North America, Geology, 40, 591–594, 2012.
Kahn, A. and Aubry, M.-P.: Provincialism associated with the Paleocene/Eocene thermal maximum: temporal constraint, Mar. Micropaleontol., 52, 117–131, https://doi.org/10.1016/j.marmicro.2004.04.003, 2004.
Katz, M. E., Pak, D. K., Dickens, G. R., and Miller, K. G.: The source and fate of massive carbon input during the Latest Paleocene Thermal Maximum, Science, 286, 1531–1533, 1999.
Kennett, J. P. and Stott, L. D.: Abrupt deep-sea warming, palaeoceanographic changes and benthic extinctions at the end of the Palaeocene, Nature, 353, 225–229, 1991.
Kent, D. V., Cramer, B. S., Lanci, L., Wang, D., Wright, J. D., and Van der Voo, R.: A case for a comet impact trigger for the Paleocene/Eocene thermal maximum and carbon isotope excursion, Earth Planet. Sc. Lett., 211, 13–26, 2003.
Kent, D. V., Lanci, L., Wang, H., and Wright, J. D.: Enhanced magnetization of the Marlboro Clay as a product of soil pyrogenesis at the Paleocene–Eocene boundary?, Earth Planet. Sc. Lett., 473, 303–312, https://doi.org/10.1016/j.epsl.2017.06.014, 2017.
Kirtland Turner, S.: Constraints on the onset duration of the Paleocene–Eocene Thermal Maximum, Philos. T. Roy. Soc. A, 376, 20170082, https://doi.org/10.1098/rsta.2017.0082, 2018.
Kirtland Turner, S. and Ridgwell, A.: Development of a novel empirical framework for interpreting geological carbon isotope excursions, with implications for the rate of carbon injection across the PETM, Earth Planet. Sc. Lett., 435, 1–13, https://doi.org/10.1016/j.epsl.2015.11.027, 2016.
Koch, P. L., Zachos, J. C., and Gingerich, P. D.: Correlation between isotope records in marine and continental carbon reservoirs near the Palaeocene/Eocene boundary, Nature, 358, 319–322, 1992.
Kopp, R. E., Schumann, D., Raub, T. D., Powars, D. S., Godfrey, L. V., Swanson-Hysell, N. L., Maloof, A. C., and Vali, H.: An Appalachian Amazon? Magnetofossil evidence for the development of a tropical river-like system in the mid-Atlantic United States during the Paleocene-Eocene thermal maximum, Paleoceanography, 24, 1–17, https://doi.org/10.1029/2009PA001783, 2009.
Li, M., Bralower, T. J., Kump, L. R., Self-Trail, J. M., Zachos, J. C., Rush, W. D., and Robinson, M. M.: Astrochronology of the Paleocene-Eocene thermal maximum on the Atlantic coastal plain, Nat. Commun., 13, 5618, https://doi.org/10.1038/s41467-022-33390-x, 2022.
Lippert, P. C. and Zachos, J. C.: A biogenic origin for anomalous fine-grained magnetic material at the Paleocene–Eocene boundary at Wilson Lake, New Jersey, Paleoceanography, 22, PA4104, https://doi.org/10.1029/2007PA001471, 2007.
Lombardi, C. J.: Lithostratigraphy and clay mineralogy of Paleocene-Eocene thermal maximum sediments at Wilson Lake, NJ, MS thesis, Rutgers University, 94 pp., 2013.
Lourens, L. J., Sluijs, A., Kroon, D., Zachos, J. C., Thomas, E., Röhl, U., Bowles, J., and Raffi, I.: Astronomical pacing of late Palaeocene to early Eocene global warming events, Nature, 435, 1083–1087, https://doi.org/10.1038/nature03814, 2005.
Lunt, D. J., Ridgwell, A., Sluijs, A., Zachos, J., Hunter, S., and Haywood, A.: A model for orbital pacing of methane hydrate destabilization during the Palaeogene, Nat. Geosci., 4, 775–778, 2011.
Makarova, M.: Application of multiproxy tracers to reconstruct paleoenvironmental perturbations on the mid-Atlantic margin across the Paleocene-Eocene thermal maximum, PhD thesis, Rutgers University, 194 pp., https://doi.org/10.7282/t3-k5md-yd57, 2018.
Makarova, M., Wright, J. D., Miller, K. G., Babila, T. L., Rosenthal, Y., and Park, J. I.: Hydrographic and ecologic implications of foraminiferal stable isotopic response across the U.S. mid-Atlantic continental shelf during the Paleocene-Eocene Thermal Maximum, Paleoceanography, 32, 1–18, https://doi.org/10.1002/2016PA002985, 2017.
McInerney, F. A. and Wing, S. L.: The Paleocene-Eocene thermal maximum: a perturbation of carbon cycle, climate, and biosphere with implications for the future, Annu. Rev. Earth Pl. Sc., 39, 489–516, 2011.
Miller, K. G., Browning, J. V., Aubry, M.-P., Babila, T., Baluyot, R. D., Esmeray-Senlet, S., Feigenson, M. D., Karakaya, S., Lombardi, C. J., McCreary, S., McLaughlin. P. P., Monteverde, D. H., Olsson, R. K., Smith C. T., Sugarman., P. J., and Wright J. D.: Wilson Lake B Site, in: Proceedings of the Ocean Drilling Program, edited by: Miller, K. G., Sugarman, P. J., Browning, J. V., McLaughlin, P. P., and Pekar, S. F., Initial reports, Vol. 174AX (Supplement), College Station, TX, https://doi.org/10.2973/odp.proc.174AXS.111.2017, 2017.
Miller, K. G., Browning, J. V., Schmelz, W. J, Kopp, R. E., Mountain, G. S., and Wright, J. D.: Cenozoic sea-level and cryospheric evolution from deep-sea geochemical and continental margin records, Science Advances, 6, eaaz1346, https://doi.org/10.1126/sciadv.aaz1346, 2020.
Mixon, R. B., Pavlides, L., Powars, D. S., Froelich, A. J., Weems, R. E., Schindler, J. S., Newell, W. L., Edwards, L. E., and Ward, L. W.: Geologic Map of the Fredericksburg 30' x 60' Quadrangle, Virginia and Maryland, U.S. Geological Survey Geologic Investigations Series Map I-2607, scale 1:100,000, 2 sheets, https://doi.org/10.3133/i2607, 2000.
Moucha, R., Forte, A. M., Mitrovica, J. X., Rowley, D. B., Quéré, S., Simmons, N. A., and Grand, S. P.: Dynamic topography and long-term sea-level variations: There is no such thing as a stable continental platform, Earth Planet. Sc. Lett., 271, 101–108, 2008.
Nittrouer, C. A., Kuehl, S. A., Figueiredo Jr., A. G., Allison, M. A., Sommerfield, C. K., Rine, J. M., Faria, L. E. C., and Silveira, O. M.: The geological record preserved by Amazon shelf sedimentation, Cont. Shelf Res., 16, 817–841, 1996.
Olsson, R. K., Miller, K. G., Browning, J. V., Habib, D., and Sugarman, P. J.: Ejecta layer at the Cretaceous-Tertiary boundary, Bass River, New Jersey (Ocean Drilling Program Leg 174AX), Geology, 25, 759–762, 1997.
Olsson, R. K., Miller, K. G., Browning, J. V., Wright, J. D., and Cramer, B. S.: Sequence stratigraphy and sea-level change across the Cretaceous-Tertiary boundary on the New Jersey passive margin, Geological Society of America Special Paper 356, 97–108, https://doi.org/10.1130/0-8137-2356-6.97, 2002.
Pagani, M., Caldeira, K., Archer, D., and Zachos, J. C.: An ancient carbon mystery, Science, 314, 1556–1557, https://doi.org/10.1126/science.1136110, 2006.
Pearson, P. N. and Nicholas, C. J.: Layering in the Paleocene/Eocene boundary of the Millville core is drilling disturbance, P. Natl. Acad. Sci. USA, 111, E1064–E1065, https://doi.org/10.1073/pnas.1322077111, 2013.
Pearson, P. N. and Thomas, E.: Drilling disturbance and constraints on the onset of the Paleocene–Eocene boundary carbon isotope excursion in New Jersey, Clim. Past, 11, 95–104, https://doi.org/10.5194/cp-11-95-2015, 2015.
Pearson, P. N., Ditchfield, P. W., Singano, J., Harcourt-Brown, K. G., Nicholas, C. J., Olsson, R. K., Shackleton, N. J., and Hall, M. A.: Warm tropical sea surface temperatures in the Late Cretaceous and Eocene epochs, Nature, 413, 481–487, https://doi.org/10.1038/35097000, 2001.
Piedrahita, V. A., Galeotti, S., Zhao, X., Roberts, A. P., Rohling, E. J., Heslop, D., Florindo, F., Grant, K. M., Rodríguez-Sanz, L., Reghellin, D., and Zeebe, R. E.: Orbital phasing of the Paleocene-Eocene Thermal Maximum, Earth Planet. Sc. Lett., 598, 117839, https://doi.org/10.1016/j.epsl.2022.117839, 2022.
Planke, S., Berndt, C., Alvarez Zarikian, C. A., and the Expedition 396 Scientists: Expedition 396 Preliminary Report: Mid-Norwegian continental margin magmatism and paleoclimate implications, International Ocean Discovery Program, https://doi.org/10.14379/iodp.pr.396.2022, 2022.
Podrecca, L. G., Makarova, M., Miller, K. G., Browning, J. V., and Wright, J. D.: Clear as mud: Expanded records of the Paleocene-Eocene Thermal Maximum onset in the Appalachian Amazon, Geology, 49, 1441–1445, https://doi.org/10.1130/G49061.1, 2021.
Robinson, M. M. and Spivey, W. E.: Environmental and geomorphological changes on the eastern North American continental shelf across the Paleocene-Eocene Boundary, Paleoceanography and Paleoclimatology, 34, 715–732, https://doi.org/10.1029/2018PA003357, 2019.
Robinson, M. M., Dowsett, H. J., and Herbert, T. D.: Very high Middle Miocene surface productivity on the U.S. mid-Atlantic shelf amid glacioeustatic sea level variability, Palaeogeogr. Palaeoecol., 606, 111249, https://doi.org/10.1016/j.palaeo.2022.111249, 2022.
Röhl, U., Westerhold, T., Bralower, T. J., and Zachos, J. C.: On the duration of the Paleocene-Eocene thermal maximum (PETM), Geochem. Geophy. Geosy., 8, Q12002, https://doi.org/10.1029/2007GC001784, 2007.
Rush, W., Kiehl, J., Shields, C., and Zachos, J.: Increased frequency of extreme precipitation events in the North Atlantic during the PETM: Observations and theory, Palaeogeogr. Palaeocl., 568, 110289, https://doi.org/10.1016/j.palaeo.2021.110289, 2021.
Rush, W., Self-Trail, J., Zhang, Y., Sluijs, A., Brinkhuis, H., Zachos, J., Ogg, J. G., and Robinson, M.: Assessing environmental change associated with early Eocene hyperthermals in the Atlantic Coastal Plain, USA, Clim. Past, 19, 1677–1698, https://doi.org/10.5194/cp-19-1677-2023, 2023.
Schaller, M. F. and Fung M. K.: The extraterrestrial impact evidence at the Palaeocene–Eocene boundary and sequence of environmental change on the continental shelf, Philos. T. Roy. Soc. A, 376, 20170081, https://doi.org/10.1098/rsta.2017.0081, 2018.
Schaller, M. F., Fung, M. K., Wright, J. D., Katz, M. E., and Kent, D. V.: Impact ejecta at the Paleocene-Eocene boundary, Science, 354, 225–229, https://doi.org/10.1126/science.aaf5466, 2016.
Schaller, M. F., Turrin, B. D., Fung, M. K., Katz, M. E., and Swisher, C. C.: Initial 40Ar-39Ar ages of the Paleocene-Eocene Boundary impact spherules, Geophys. Res. Lett., 46, 9091–9102, https://doi.org/10.1029/2019GL082473, 2019.
Schmelz, W. J., Miller, K. G., Kopp, R., Mountain, G. S., and Browning, J. V.: Influence of mantle dynamic topographical variations on US mid-Atlantic continental margin estimates of sea-level change, Geophys. Res. Lett., 48, e2020GL090521, https://doi.org/10.1029/2020GL090521, 2021.
Schmelz, W. J, Miller, K. G., Mountain, G. S., Steckler, M. S., Kopp, R. E., and Browning, J. V.: Sensitivity of modeled passive margin stratigraphy to variations in sea level, sediment supply, and subsidence, Basin Res., 36, e12854, https://doi.org/10.1111/bre.12854, 2024.
Schulte, P., Alegret, L., Arenillas I., Arz, J. A., Barton, P. J., Bown, P. R., Bralower, T. J., Christeson, G. L., Claeys, P., Cockell, C. S., Collins, G. S., Deutsch, A., Goldin, T. J., Goto, K., Grajales-Nishimura, J. M., Grieve, R. A. F., Gulick, S. P. S., Johnson, K. R., Kiessling, W., Koeberl, C., Kring, D. A., Macleod, K. G., Matsui, T., Melosh, J., Montanari, A., Morgan, J. V., Neal, C. R., Nichols, D. J., Norris, R. D., Pierazzo, E., Ravizza, G., Rebolledo-Vieyra, M., Reimold, W. U., Robin, E., Salge, T., Speijer, R. P., Sweet, A. R., Urrutia-Fucugauchi, J., Vajda, V., Whalen, M. T., and Willumsen, P. S.: The Chicxulub asteroid impact and mass extinction at the Cretaceous-Paleogene boundary, Science, 327, 1214–1218, https://doi.org/10.1126/science.1177265, 2010.
Secord, R., Gingerich, P. D., Lohmann, K. C., and MacLeod, K. G.: Continental warming preceding the Palaeocene-Eocene thermal maximum, Nature, 467, 955–958, https://doi.org/10.1038/nature09441, 2010.
Self-Trail, J. M., Hajek, E. A., Edwards, L. E., Robinson, M. M., Bralower, T. J., Sessa, J. A., Kump, L. R., Trampush, S. M., Willard, D. A., Powars, D. S., and Wandless, G. A.: Shallow marine response to global climate change during the Paleocene-Eocene Thermal Maximum, Salisbury Embayment, USA, Paleoceanography, 32, 710–728, https://doi.org/10.1002/2017PA003096, 2017.
Setty, S., Cramwinckel, M. J., van Nes, E. H., van de Leemput, I. A., Dijkstra, H. A., Lourens, L. J., Scheffer, M., and Sluijs, A.: Loss of Earth system resilience during early Eocene transient global warming events, Science Advances, 9, eade5466, https://doi.org/10.1126/sciadv.ade5466, 2023.
Si, W. and Aubry, M.-P.: Vital effects and ecologic adaptation of photosymbiont-bearing planktonic foraminifera during the Paleocene-Eocene Thermal Maximum, implications for paleoclimate, Paleoceanography and Paleoclimatology, 33, 1–14, https://doi.org/10.1002/2017PA003219, 2018.
Sluijs, A., Schouten, S., Pagani, M., Woltering, M., Brinkhuis, H., Sinninghe Damsté, J. S., Dickens, G. R., Huber, M., Reichart, G.-J., Stein, R., Matthiessen, J., Lourens, L. J., Pedentchouk, N., Backman, J., Moran, K., and the Expedition 302 Scientists: Subtropical Arctic Ocean temperatures during the Palaeocene/Eocene thermal maximum, Nature, 441, 610–613, https://doi.org/10.1038/nature04668, 2006.
Sluijs, A., Brinkhuis, H., Schouten, S., Bohaty, S. M., John, C. M., Zachos, J. C., Reichart, G.-J., Sinninghe Damsté, J. S., Crouch, E. M., and Dickens, G. R.: Environmental precursors to rapid light carbon injection at the Palaeocene/Eocene boundary, Nature, 450, 1218–1221, https://doi.org/10.1038/nature06400, 2007.
Sluijs, A., Brinkhuis, H., Crouch, E. M., John, C. M., Handley, L., Munsterman, D., Bohaty, S. M., Zachos, J. C., Reichart, G.-J., Schouten, S., Pancost, R. D., Sinninghe Damsté, J. S., Welters, N. L. D., Lotter, A. F., and Dickens, G. R.: Eustatic variations during the Paleocene-Eocene greenhouse world, Paleoceanography and Paleoclimatology, 23, PA4216, https://doi.org/10.1029/2008PA001615, 2008.
Sluijs, A., Schouten, S., Donders, T. H., Schoon, P. L., Röhl, U., Reichart, G.-J., Sangiorgi, F., Kim, J.-H., Sinninghe Damste, J. S., and Brinkhuis, H.: Warm and wet conditions in the Arctic region during the Eocene Thermal Maximum 2, Nat. Geosci., 11, 777–780, https://doi.org/10.1038/ngeo668, 2009.
Stassen, P., Thomas, E., and Speijer, R. P.: Integrated stratigraphy of the Paleocene-Eocene thermal maximum in the New Jersey Coastal Plain: Toward understanding the effects of global warming in a shelf environment, Paleoceanography, 27, 1–17, https://doi.org/10.1029/2012PA002323, 2012.
Stassen, P., Thomas, E., and Speijer, R. P.: Paleocene–Eocene Thermal Maximum environmental change in the New Jersey Coastal Plain: benthic foraminiferal biotic events, Mar. Micropaleontol., 115, 1–23, https://doi.org/10.1016/j.marmicro.2014.12.001, 2015.
Steckler, M. S., Mountain, G. S., Miller, K. G., and Christie-Blick, N.: Reconstruction of Tertiary progradation and clinoform development on the New Jersey passive margin by 2-D backstripping, Mar. Geol., 154, 399–420, 1999.
Thomas, D. J., Bralower, T. J., and Zachos, J. C.: New evidence for subtropical warming during the late Paleocene thermal maximum: Stable isotopes from Deep Sea Drilling Project Site 527, Walvis Ridge, Paleoceanography, 14, 561–570, 1999.
Thomas, E.: Development of Cenozoic deep-sea benthic foraminiferal faunas in Antarctic waters, Geological Society London Special Publication, 47, 283–296, 1989.
Thomas, E. and Zachos, J. C.: Was the late Paleocene thermal maximum a unique event?, Geologiska Föreningens i Stockholm Förhandlingar (GFF; Transactions of the Geological Society in Stockholm), 122, 169–170, 2000.
Tian, S. Y., Yasuhara, M., Robinson, M. M., and Huang, H.-H. M.: Ostracod eye size: A taxonomy-free indicator of the Paleocene-Eocene Thermal Maximum sea level, Mar. Micropaleontol., 174, 101994, https://doi.org/10.1016/j.marmicro.2021.101994, 2022.
Tierney, J. E., Zhu, J., Li, M., and Kump, L. R.: Spatial patterns of climate change across the Paleocene-Eocene Thermal Maximum, P. Natl. Acad. Sci. USA, 119, e2205326119, https://doi.org/10.1073/pnas.2205326119, 2022.
Tripati, A. K. and Elderfield, H.: Abrupt hydrographic changes in the equatorial Pacific and subtropical Atlantic from foraminiferal indicate greenhouse origin for the thermal maximum at the Paleocene-Eocene Boundary, Geochem. Geophy. Geosy., 5, Q02006, https://doi.org/10.1029/2003GC000631, 2004.
Vimpere, L., Spangenberg, J., Roige, M., Adatte, T., De Kaenel, E., Fildani, A., Clark, J., Sahoo, S., Bowman, A., Sternai, P., and Castelltort, S.: Carbon isotope and biostratigraphic evidence for an expanded Paleocene–Eocene Thermal Maximum sedimentary record in the deep Gulf of Mexico, Geology, 51, 334–339, https://doi.org/10.1130/G50641.1, 2023.
Wang, H., Kent, D. V., and Jackson, M. J.: Evidence for abundant isolated magnetic nanoparticles at the Paleocene–Eocene boundary, P. Natl. Acad. Sci. USA, 110, 425–430, https://doi.org/10.1073/pnas.1205308110, 2013.
Westerhold, T., Röhl, U., Frederichs, T., Agnini, C., Raffi, I., Zachos, J. C., and Wilkens, R. H.: Astronomical calibration of the Ypresian timescale: implications for seafloor spreading rates and the chaotic behavior of the solar system?, Clim. Past, 13, 1129–1152, https://doi.org/10.5194/cp-13-1129-2017, 2017.
Westerhold, T., Röhl, U., Donner, B., and Zachos, J. C.: Global extent of Early Eocene hyperthermal events: A new Pacific benthic foraminiferal isotope record from Shatsky Rise (ODP Site 1209), Paleoceanography and Paleoclimatology, 33, 626–642, https://doi.org/10.1029/2017PA003306, 2018.
Wing, S. L., Harrington, G. J., Smith, F. A., Bloch, J. I., Boyer, D. M., and Freeman, K. H.: Transient floral change and rapid global warming at the Paleocene-Eocene boundary, Science, 310, 993–996, https://doi.org/10.1126/science.1116913, 2005.
Wright, J. D. and Schaller, M. F.: Evidence for a rapid release of carbon at the Paleocene-Eocene Thermal Maximum, P. Natl. Acad. Sci. USA, 110, 15908–15913, https://doi.org/10.1073/pnas.1309188110, 2013.
Zachos, J. C., Wara, M. W., Bohaty, S. M., Delaney, M. L., Petrizzo, M. R., Brill, A., Bralower, T. J., and Premoli-Silva, I.: A transient rise in tropical sea surface temperature during the Paleocene-Eocene thermal maximum, Science, 302, 1551–1554, https://doi.org/10.1126/science.1090110, 2003.
Zachos, J. C., Röhl, U., Schellenberg, S. A., Sluijs, A., Hodell, D. A., Kelly, D. C., Thomas, E., Nicolo, M., Raffi, I., Lourens, L. J., McCarren, H., and Kroon, D.: Rapid Acidification of the Ocean During the Paleocene-Eocene Thermal Maximum, Science, 308, 1611–1615, https://doi.org/10.1126/science.1109004, 2005.
Zachos, J. C., Bohaty, S. M., John, C. M., McCarren, H., Kelly, D. C., and Nielsen, T.: The Palaeocene–Eocene carbon isotope excursion: constraints from individual shell planktonic foraminifer records, Philos. T. Roy. Soc. A, 365, 1829–1842, https://doi.org/10.1098/rsta.2007.2045, 2007.
Zachos, J. C., McCarren, H., Murphy, B., Röhl, U., and Westerhold, T.: Tempo and scale of late Paleocene and early Eocene carbon isotope cycles: Implications for the origin of hyperthermals, Earth Planet. Sc. Lett., 299, 242–249, https://doi.org/10.1016/j.epsl.2010.09.004, 2010.
Zeebe, R. E. and Lourens, L. J.: Solar system chaos and the Paleocene-Eocene boundary age constrained by geology and astronomy, Science, 365, 926–929, https://doi.org/10.1126/science.aax0612, 2019.
Zeebe, R. E., Ridgwell, A., and Zachos, J. C.: Anthropogenic carbon release rate unprecedented during the past 66 million years, Nat. Geosci., 9, 325–329, https://doi.org/10.1038/ngeo2681, 2016.
Zhou, X., Thomas, E., Rickaby, R. E. M., Winguth, A. M. E., and Lu, Z.: evidence for upper ocean deoxygenation during the PETM, Paleoceanography, 29, 964–975, https://doi.org/10.1002/2014PA002702, 2014.
- Abstract
- Introduction
- US mid-Atlantic Coastal Plain sediments
- Existing PETM climate records from the US mid-Atlantic Coastal Plain
- Proposed drill sites
- Drilling plan challenges
- Shallow marine records of Paleocene to Eocene global climate and ecosystem perturbations – research approach and science questions
- Summary
- Data availability
- Author contributions
- Competing interests
- Disclaimer
- Acknowledgements
- Review statement
- References
- Abstract
- Introduction
- US mid-Atlantic Coastal Plain sediments
- Existing PETM climate records from the US mid-Atlantic Coastal Plain
- Proposed drill sites
- Drilling plan challenges
- Shallow marine records of Paleocene to Eocene global climate and ecosystem perturbations – research approach and science questions
- Summary
- Data availability
- Author contributions
- Competing interests
- Disclaimer
- Acknowledgements
- Review statement
- References