the Creative Commons Attribution 4.0 License.
the Creative Commons Attribution 4.0 License.
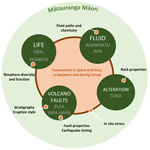
CALDERA: a scientific drilling concept to unravel Connections Among Life, geo-Dynamics and Eruptions in a Rifting Arc caldera, Okataina Volcanic Centre, Aotearoa New Zealand
Ludmila Adam
Eric S. Boyd
S. Craig Cary
Daniel R. Colman
Alysia Cox
Ery Hughes
Geoff Kilgour
Matteo Lelli
Domenico Liotta
Karen G. Lloyd
Tiipene Marr
David D. McNamara
Sarah D. Milicich
Craig A. Miller
Santanu Misra
Alexander R. L. Nichols
Simona Pierdominici
Shane M. Rooyakkers
Douglas R. Schmitt
Andri Stefansson
John Stix
Matthew B. Stott
Camille Thomas
Pilar Villamor
Pujun Wang
Sadiq J. Zarrouk
Silicic caldera volcanoes present major volcanic and seismic hazards but also host dynamic hydrothermal and groundwater systems and a rich but largely unexplored subsurface biosphere. Many of these volcanoes are hosted in rift settings. The intricate connections and feedbacks among magmatism, rifting, hydrothermal processes, and the biosphere in these complex systems remain poorly understood, necessitating subsurface joint observations that are only enabled by scientific drilling. The CALDERA (Connections Among Life, geo-Dynamics and Eruptions in a Rifting Arc caldera) project workshop funded by the International Continental Scientific Drilling Program (ICDP) gathered multi-disciplinary international experts in January 2023 to advance planning of a scientific drilling project within one of these dynamic, rift-hosted calderas, the Okataina Volcanic Centre (OVC), Aotearoa New Zealand.
The OVC's high eruption rate, frequent unrest events and earthquake swarms, location in a densely faulted rapidly extending rift, abundant groundwater–geothermal fluid circulations, and diverse surface hot spring microbiota make it an ideal location for exploring a connected geo-hydro-biosphere via scientific drilling and developing a test bed for novel volcano monitoring approaches. Drilling configurations with at least two boreholes (∼ 200 and ∼ 1000–1500 m deep) were favoured to achieve the multi-disciplinary objectives of the CALDERA project. Decadal monitoring including biosphere activity and composition has the potential to evaluate the response of the hydro-bio system to volcano-tectonic activity. In addition to the OVC caldera-scale datasets already available, site surveys will be conducted to select the best drilling locations.
The CALDERA project at the OVC would provide, for the first time, an understanding of volcanic–tectonic–hydrological–biological connections in a caldera–rift system and a baseline for global comparisons with other volcanoes, rifts, and hydrothermal systems. CALDERA would serve as an unprecedented model system to understand how and how quickly the subsurface biosphere responds to geologic activities. Discoveries will improve assessment of volcanic and seismic hazards, guide the sustainable management and/or conservation of groundwater and geothermal resources and microbial ecosystems, and provide a forum for interweaving mātauranga Māori and Western knowledge systems.
- Article
(5886 KB) - Full-text XML
-
Supplement
(514 KB) - BibTeX
- EndNote
Karakia Whakawātea
Unuhia unuhia
Unuhia te urutapu nui kia wātea
Kia māmā te ngākau, te tinana me te wairua
Koia rā e Rongo, whakairia ake ki runga
Kia tīna! Tīna
Haumi e, Hui e, Tāiki e
Draw away, draw away
Draw away the sacredness and let us be free
Let our hearts, bodies and spirit be unburdened
Oh Rongo suspend this plea up high
And let it be affirmed
Let it be binding, together, all in agreement
As per Māori custom, we start and end this paper (kōrero) with a karakia provided in Te Reo Māori for CALDERA. A karakia is a prayer of respect and thanks to Papatūānuku our Earth mother and sky father Ranginui, as well as to the taiao (environment) that gives us life.
Caldera–rift settings are ideal for unravelling the connections between volcanic, tectonic, hydrologic, and microbial processes, both between and during episodes of volcanic or tectonic unrest, which are poorly understood (Fig. 1). Caldera volcanoes generate the largest explosive eruptions on Earth by rapidly emptying their magma reservoir in association with earthquakes (Self, 2006). However, the geological drivers of caldera eruptions and earthquakes, their size, their interactions, and their timescales are poorly understood (Hilley et al., 2022). This limits our ability to discern whether signals of activity (e.g. seismicity and ground deformation) are due to volcanic unrest, imminent eruption, or solely tectonic rifting. The combination of a large magmatic heat source and crustal fracturing (e.g. caldera collapse, rift faults, and fracture networks) results in dynamic and complex fluid circulations that need to be better understood to sustainably use geothermal and groundwater resources. In hydrothermal settings, little is known about subsurface microbial diversity and how the function and structure of microbial communities react to changes in subsurface processes, either natural (e.g. groundwater recharge, earthquakes, or volcanic activity) or from utilisation of resources (e.g. geothermal or groundwater) (Cluff et al., 2014; Mu and Moreau, 2015). Also unknown are the effects that these changes may have on surface ecosystems (e.g. modulation of greenhouse gases and fluid chemistry).
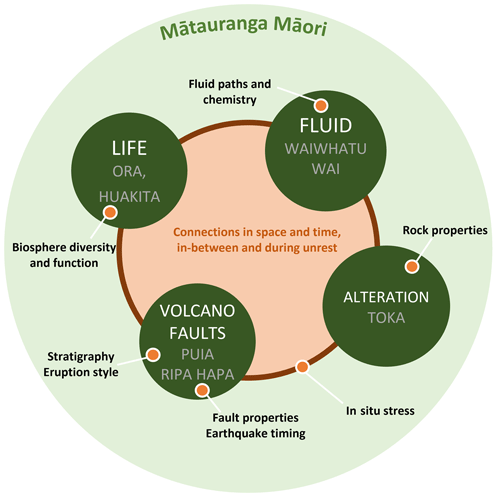
Figure 1Overview of CALDERA: topics (orange dots) along connected themes (dark-green circles, with a Te Reo Māori language equivalent). Mātauranga Māori (Indigenous knowledge) is intended to be embedded throughout the project.
Multiple feedbacks observed between processes in caldera–rift settings have not been fully reconciled. Faulting and deep basaltic injection, both enhanced by rifting, alter crustal stresses, pore pressures, and magma body overpressures, in some cases triggering voluminous silicic caldera-forming eruptions (Hughes and Mahood, 2011; Allan et al., 2012; Morgavi et al., 2017; Zhan and Gregg, 2019). Fault geometries and activities depend on in situ stress at various temporal and spatial scales, fluid pressure and composition, and rock properties. In turn, rock properties depend on the primary rock type and evolving water–rock interactions. Fluid pathways and compositions are complex due to variable lithologies, hydrothermal alteration, faults, and stress fields, all modulated by volcano-tectonic activity (Wilson and Rowland, 2016). The circulation of mixed hydrothermal and cold meteoric fluids affects the thermal and mechanical states of the crust, which in turn influence the thresholds for both magma chamber failure and fault rupture (Jolie et al., 2016). The deep biosphere composition and activity depend on fluid and rock compositions (Gold, 1992). Conversely, subsurface microorganisms modify rock and fluid properties, likely creating feedback loops traversing deep and surficial processes.
Understanding these connected processes is important for informing strategies to (1) increase resilience to geohazards and (2) adapt to climate change by sustainably conserving and managing geothermal and groundwater resources and their ecosystems, developing biotechnologies, and better understanding elemental cycling for greenhouse gas budgets. Views from local communities, especially Indigenous groups that are closely related to the land (through ancestry, spirituality, use of resources, and wellbeing), are key to developing robust strategies alongside scientific findings.
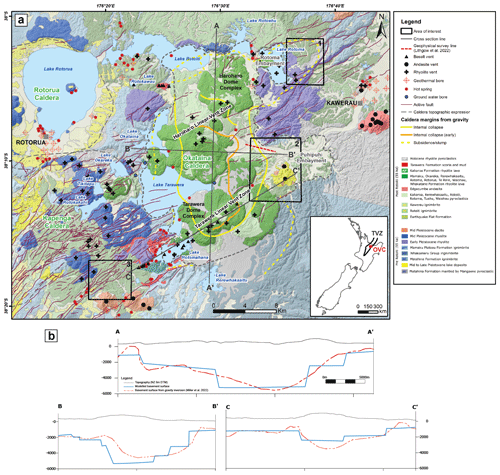
Figure 2(a) Geology of the OVC, location of the active faults (New Zealand Active Faults Database, 2003), structural (as defined from gravity, Miller et al., 2022b) and topographic margins, geothermal and groundwater wells, geophysical survey line (Lithgow et al., 2022; see Fig. 5), and proposed drilling areas. The inset shows the location of the OVC and TVZ in Aotearoa New Zealand. (b) Cross sections through the OVC 3-D model showing the basement surface from gravity inversion (Miller et al., 2022b) and geologically modelled (Carson et al., 2022).
Multi-disciplinary data arising from scientific drilling have high potential to unravel geo-hydro-bio processes in caldera–rift settings. The Okataina Volcanic Centre (OVC) in the Taupō Volcanic Zone (TVZ), Aotearoa New Zealand, is ideally suited for such an endeavour because it is one of Earth's most active calderas, it interacts with the rapidly extending Taupō Rift, and it hosts active groundwater–geothermal systems and a varied biosphere in hot springs (Fig. 2). Insights made by scientific drilling at the OVC will support understanding of those connected processes worldwide.
This paper summarises the outcomes of an international workshop that advanced planning of the Connections Among Life, geo-Dynamics and Eruptions in a Rifting Arc caldera (CALDERA) scientific drilling concept. Supported by the International Continental Scientific Drilling Program (ICDP), the workshop resulted in an interdisciplinary science plan. In this paper, we start by presenting the OVC and the goal of interweaving mātauranga Māori (Indigenous knowledge) in the project. Then, we articulate CALDERA's research aims and how they address global societal challenges. Finally, we summarise strategies for successfully conducting the project.
1.1 The Okataina Volcanic Centre, Aotearoa New Zealand: a landmark system
The OVC is ideally positioned to address global scientific and societal needs. The OVC is one of two giant active calderas in the TVZ, located within the major active fault system of the Taupō Rift (Cole et al., 2014). The OVC is ranked as Aotearoa New Zealand's highest threat volcano (Miller and Jolly, 2014; Miller et al., 2022a).
The OVC is one of the most frequently active rhyolite volcanic centres on Earth (> 150 km3 of magma erupted over the last ∼ 50–60 000 years; Nairn, 2002; Wilson et al., 2009), driven by high rates of mantle melting and rapid intra-arc continental rifting (Taupō Rift) (Houghton et al., 1995; Wilson et al., 1995; Nairn, 2002; Cole et al., 2014; Villamor et al., 2017a; Barker et al., 2020). During its known ∼ 625 kyr volcanic history, the OVC has experienced two definite caldera-forming eruptions (Matahina, ∼ 322 ka, and Rotoiti, 50–60 ka). Additional caldera subsidence may have occurred during eruption of the Utu/Quartz-Biotite (∼ 557 ka) and Kawerau (∼ 33 ka) ignimbrites (Nairn, 2002; Cole et al., 2014; Miller et al., 2022b). These major events bracket numerous smaller intra-caldera explosive events and dome-building episodes (e.g. Nairn, 2002). Plinian-scale basalt and rhyolite eruptions have occurred from the same vent systems (Nairn, 2002; Cole et al., 2014), yielding opportunities to sample varied materials from a single location. The latest OVC eruption occurred in 1886 AD at Tarawera Maunga (mountain). Although of a small size for the OVC, the 1886 basaltic eruption cost the lives of ∼ 120 people and heavily impacted local Māori (Rowe et al., 2021). Numerous examples of paleo-earthquakes, in certain cases associated with volcanic eruptions, have been documented on active faults of the Taupō Rift just outside the OVC (Berryman et al., 2022; Villamor et al., 2022). The OVC region is seismically active and has experienced three seismic swarms in the past 15 years (Benson et al., 2021; Bannister et al., 2022).
The heat from several hydrothermal systems outflowing on the edges of the caldera is inferred to come from the deep magmatic complex in the centre of the caldera (Bertrand et al., 2022). Magmatic fluids interact with abundant freshwater, including groundwater, rivers, lakes, and rainfall of 1.5–2 m yr−1 (Giggenbach, 1995; Mazot et al., 2014; Simpson and Bignall, 2016; Hughes et al., 2019; Pearson-Grant et al., 2022; Yang et al., 2023). Diverse microbiota have been identified in the caldera and rift-straddling Waimangu geothermal field (Power et al., 2018, 2024). The absence of geothermal fluid extraction makes the OVC a rare pristine system largely unaltered by human activities.
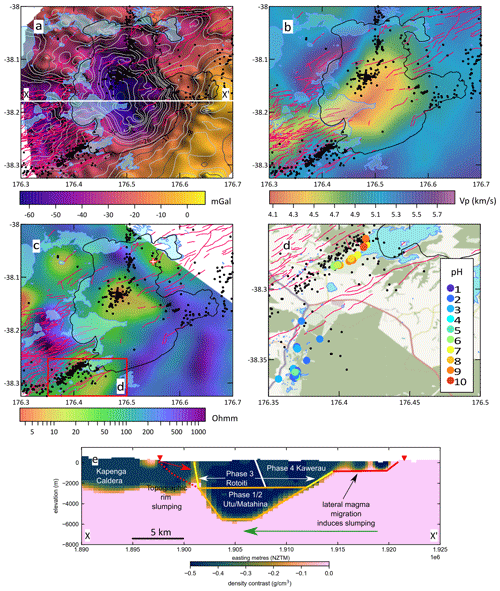
Figure 3Regional geophysical data around the OVC suggesting a magma reservoir at > 7 km, known active faults (red lines in panels (a)–(d)), topographical margin of the OVC (black line), and relocated seismicity (black dots). (a) Residual gravity anomaly (Miller et al., 2022b). (b) P-wave velocity at 3 ± 2 km depth (Bannister et al., 2022). (c) Magnetotelluric resistivity model at 4 km (Bertrand et al., 2022). (d) pH of some of the hydrothermal features sampled for the fluid chemistry and biosphere during the 1000-spring project in a subset area of the OVC (Power et al., 2018). (e) Cross section with inferred structures from gravity (extent in panel (a)).
The OVC has been extensively studied from the surface for decades (Fig. 3, Table S1 in the Supplement). This will enable integration of detailed borehole data in the regional and local contexts to generate transformational research. The record of eruptions since the last caldera-forming event (50–60 ka) is very well documented (e.g. Nairn, 2002) and will allow correlations across boreholes, but small eruptions may still be missing, and the older record is incomplete. The volcano-tectonic paleoseismic record is possibly the best in the world. Natural hazard monitoring data (seismicity, geodetics, and chemistry) collected by the GeoNet programme are publicly available.
1.2 Mātauranga Māori (Indigenous knowledge)
Indigenous knowledge systems are based on intergenerational holistic observation and location-based experience. They are founded on the same principles of hypothesis testing and re-evaluation that underpin contemporary Earth and biological sciences (Lazrus et al., 2022). In Aotearoa New Zealand, the Māori Indigenous Knowledge System is referred to as mātauranga. Mātauranga is defined as the “pursuit and application of knowledge and understanding of te taiao [the environment], following a systematic methodology based on evidence, incorporating culture, values and world views” (Hikuroa, 2017). The holistic approach in mātauranga that includes connections between all aspects of te taiao has great potential to enrich the project outcomes (e.g. Taute et al., 2022).
It is a goal of the CALDERA project to co-design the project with mana whenua (Māori that hold rangatiratanga – sovereignty) and to interweave mātauranga through the lifecycle of the project to ensure the research serves both mana whenua and scientists. Our aim is that mana whenua will be able to contribute to, and directly benefit from, the research findings and their impacts on resilience to hazards, sustainable management of resources and ecosystems, and biotechnology development potential. A bi-cultural outreach programme will particularly benefit rangatahi (the young generation) to support them in becoming next-generation thought leaders.
1.3 Workshop organisation
The CALDERA workshop was held on 24–27 January 2023. Over 40 scientists from 13 countries attended the workshop at Tauranga, Waikato University Campus, Aotearoa New Zealand. An additional nine scientists from four countries participated online. The group covered all disciplines of the project and career stages including students and early-career and senior scientists. Representatives from local government (Bay of Plenty Regional Council, Waikato Regional Council) and drillers (Webster Drilling, Contact Energy Ltd) also contributed to parts of the workshop.
The workshop started with a mihi whakatau (Māori welcome ceremony) by the kaumātua (elder) of the Waikato University campus. Tiipene Marr (Te mana o te Ngāti Rangitihi) introduced the participants to some historical and cultural Māori aspects of the region, followed by a question-and-answer session. Māori aspects were further discussed throughout the workshop, including during the field trip to the Lake Tarawera, Paeroa Fault, and Waimangu geothermal systems.
Prior to the workshop, participants were asked to prioritise the research questions proposed in the ICDP workshop proposal through an online form. This facilitated discussions during the workshop towards addressing recommendations from the ICDP review panel. About two-thirds of the time was dedicated to discussions in breakout groups of single- or cross-discipline and full-group discussions. Opportunities for sharing of knowledge between local Māori and the science team, as well as general education and outreach activities, were discussed. Numerous workshop participants have already conducted outreach activities, including with Indigenous communities, which will facilitate the development of a compelling outreach programme.
2.1 CALDERA aims
The goal of CALDERA is to illuminate the spatiotemporal connections and feedbacks between volcanic, tectonic, hydrological, and biological processes that are especially clear in a caldera–rift setting. The project will advance the grand challenge of resolving the factors underpinning the distribution, abundance, diversity, and activity of deep subsurface biospheres. Associated monitoring data will reveal how and how quickly the system responds to volcanic and tectonic unrest. The project will involve mātauranga Māori for a holistic understanding.
The multi-disciplinary objectives encompass three overarching research topics that will be investigated through scientific drilling to provide a step change in the understanding of
-
the build-up to eruptions of different sizes, including caldera-forming eruptions using the past volcano-tectonic interactions and pre-caldera-forming volcano stratigraphy;
-
how fluid properties and pathways are modulated by volcano-tectonic processes, which influence hydrothermal alteration through time; and how monitoring of fluid property changes contributes to interpreting signals of volcano-tectonic unrest; and
-
how subsurface microorganisms are varied in composition, diversity, and function and sensitively respond to volcano-tectonic activity. The CALDERA project will test whether subsurface microorganisms could serve as early indicators of volcano and/or tectonic events.
Datasets collected during CALDERA will also address a wide range of secondary objectives and contribute to global topics such as volcanic rift basin initiation in active continental margins, rift and volcanic arc evolution, and formation of ore deposits.
2.2 Topic 1: volcanoes, tectonics, and their interactions
Understanding how, when, and why calderas erupt remains among the greatest challenges in Earth sciences because calderas are intrinsically challenging to study, understand, and monitor (Wilson et al., 2021; Sparks et al., 2022). Firstly, deposits from their early eruptions are usually deeply buried or destroyed by later catastrophic eruptions and subsidence, so little is known about the nature, timing, and size of early caldera eruptions or how their magmatic systems mature and evolve towards catastrophic events (Bouvet de Maisonneuve et al., 2021). Secondly, caldera volcanoes are famously restless, but the origins of the unrest are often equivocal, and most unrest episodes do not culminate in eruptions (e.g. Sparks, 2003; Lowenstern et al., 2006; Acocella et al., 2016; Costa, 2021; Illsley-Kemp et al., 2021; Scarpa et al., 2022). Thirdly, caldera behaviour is complex, as it is modulated by interactions between local (volcano-related) stresses and regional stresses and deep magmatic processes that are difficult to disentangle and that may involve complex and non-linear feedbacks (e.g. Bursik et al., 2003; Rowland et al., 2010; Allan et al., 2012; Cabaniss et al., 2018; Muirhead et al., 2022).
Expanding the detailed records of past eruptions is needed to interpret past volcano-tectonic interaction events and help assess tipping points for caldera unrest. Causal relationships between individual earthquakes and volcanic eruptions have been documented over distances that can reach up to hundreds of kilometres but more commonly tens of kilometres (e.g. Linde and Sacks, 1998; Hill et al., 2002; Marzocchi, 2002). The prehistoric record of triggering of volcanic eruptions by large earthquakes and vice versa is world-class at the OVC but still likely incomplete (e.g. Bursik et al., 2003; Berryman et al., 2008, 2022; Muirhead et al., 2022; Villamor et al., 2011, 2022). Historic examples of caldera eruptions are scarce (Hildreth, 1991; Abe, 1992), and the role or significance of large earthquakes within the unrest sequence is difficult to discern in the absence of detailed unrest monitoring.
Crustal structure and magma dynamics are intimately linked at all scales, but how this interaction occurs remains poorly constrained (Walter and Amelung, 2007; Mahony et al., 2011; Villamor et al., 2017a; Oliva et al., 2019). The weakening of the crust by a magma reservoir influences the location, style, and rates of activity of faults in colder areas of the adjacent rift (van Wyk de Vries and Merle, 1996; Lahitte et al., 2003; Ellis et al., 2014; Villamor et al., 2017a, 2022), yet modelling relies on incomplete data (Corti, 2012). Fault rupture occurs due to increased stresses and/or fluid pressure on the fault plane (Byerlee and Savage, 1992). While stresses from magma dynamics and fault ruptures have been quantified for decades (Stein, 1999; Ruz-Ginouves et al., 2021), changes in fluid pressure during these processes are difficult to quantify without direct measurements.
Accessing the subsurface of caldera–rift systems via scientific drilling is needed to address these challenges by (1) sampling thin or deeply buried early eruption deposits to complete eruption histories, understand volcanic evolution prior to a large caldera-forming event, and infer influences on rift evolution; (2) revealing the internal rock composition, structure, and properties of fault zones (e.g. strength, permeability); (3) measuring in situ stresses; and (4) monitoring strain, seismicity, and fluid properties to guide interpretation of unrest. Downhole monitoring of faults near the caldera, together with regional volcano-tectonic monitoring and detailed understanding of past events, is needed to understand the faults' changing conditions caused by volcanic unrest, rifting, or both. These will contribute to assessing whether unrest may lead to an eruption, a large earthquake, or both.
2.3 Topic 2: fluid properties, pathways, and water–rock interactions
2.3.1 Hydrology, fluid properties, and structural permeability
The origin and chemistry of fluids in magmatic–hydrothermal systems are complex. Deep fluids at lithostatic pressure near the magmatic heat source (superheated or supercritical fluid, saline or hypersaline brines rich in volatiles) mix with meteoric waters to yield fluids in the liquid or vapour phase, circulating at shallow levels at or below hydrostatic pressure. Conceptual models of hydrothermal systems suggest that the low-permeability zone around the brittle–ductile transition is episodically penetrated by magmatic volatiles in response to stress and strain changes (e.g. Hayba and Ingebritsen, 1997; Fournier, 1999; Scott et al., 2015). Validating these models and investigating the continuous interplay among magmatic and geothermal fluids and freshwater require monitoring of the chemistry of subsurface hydrothermal fluids that reflect magmatic inputs in a pristine system not modified by geothermal energy developments.
Fluid flow paths in caldera–rift systems are strongly controlled by fracture networks (e.g. Arnórsson, 1995; Norini et al., 2019; Rowland and Simmons, 2012) and typically difficult to evaluate. Fracture networks are determined by competing factors that are insufficiently understood individually and in conjunction: (1) spatiotemporal connections between rift and caldera fault systems among regional fractures (Acocella, 2014; Villamor et al., 2017a); (2) fracture kinematics with respect to crustal stress states (Sibson, 2000) and connectivity among fractures (Sanderson and Nixon, 2015); (3) fluid–rock interaction processes that vary with fluid pressure and chemistry (McNamara et al., 2016; Zucchi et al., 2017; Uno et al., 2022); (4) in situ stress variations arising from fluid-pressure changes (sealing during progressive magma cooling) and gravitational collapses (Somr et al., 2023); and (5) repeated volcanic and tectonic events that maintain fluid circulations from deep to shallow (Shapiro et al., 2017). Volcanic and lake sediments also contribute to a heterogeneous permeability within calderas that is difficult to assess from the surface. Ground deformation caused by volcanic and rifting processes also influences the fracture network connectivity and fluid conditions (Battaglia et al., 2006; Gottsmann et al., 2007; Hutnak et al., 2009).
Only co-located measurements of rock and fracture properties, in situ stress, and fluid compositions and pathways can clarify connections among the factors that drive fault activity and permeability. Caldera–rift systems are ideal for exploring these unresolved processes because they host intense fluid circulations driven by large heat sources and with faults that are often reactivated. Drilling is required to (1) identify flow paths and quantify the mixing between meteoric recharge (including multi-layer aquifers) and deep magmatic–hydrothermal fluids, including in response to volcanic unrest or fault activity; (2) access depths where fluids are less or not affected by surface water bodies and shallow groundwater; and (3) obtain cores to characterise the fracture network and its evolution through past volcano-tectonic events. Downhole monitoring is the best way to identify changes in fluid composition in response to volcanic or tectonic activity. These subsurface data are needed to parametrise hydro-mechanical models, with implications for biosphere studies.
2.3.2 Volcanic rock properties and evolution of hydrothermal alteration
Volcanic rock properties underpin the understanding of volcanic and hydrothermal systems. These fundamental datasets and concepts are needed to interpret geophysical surveys and calibrate models of deformation (faulting, caldera collapse), hydrology, and how mineral composition affects the deep biosphere. In turn, these models are needed to interpret signals of tectonic and volcanic activity. Hydrothermal alteration types and kinematics are driven by the combination of (1) host rock mineralogy; (2) subsurface fluid chemistry; (3) fluid pathways; and (4) pressure and temperature conditions. The interpretation of the evolution of hydrothermal alteration and how it changes volcanic rock properties is insufficiently constrained globally due to the scarcity of multi-scale borehole data.
The evolution of hydrothermal alteration has contrasting and variable effects on volcanic rock properties. Argillic alteration can alternately increase porosity and weaken rocks (Wyering et al., 2014) or decrease permeability and strengthen rocks (Mordensky et al., 2018; Nicolas et al., 2020). Acid–sulfate alteration on andesitic rocks can increase (Kennedy et al., 2020; Kanakiya et al., 2021a) or decrease (Heap et al., 2019) porosity and permeability. The original rock microstructure, especially porosity and permeability, influences hydrothermal alteration (Mordensky et al., 2019; Heap and Violay, 2021), but the original volcanic rock properties have been characterised for only a few lithologies sampled at different stratigraphic intervals or localities (Pola et al., 2014; Mordensky et al., 2019; Kanakiya et al., 2021a). Laboratory experimental studies have primarily focused on the influence of hydrothermal alteration upon fluid pathway evolution (Pola et al., 2014; Wyering et al., 2014), rheology and strength (Heap and Violay, 2021), elastic wave velocities (Kanakiya et al., 2021a), resistivity (Komori et al., 2013), and magnetisation (Kanakiya et al., 2021b). Extrapolating to the field scale to understand how hydrothermal alteration influences deformation and geophysical signatures requires in situ microscale-to-macroscale data.
Scientific drilling is the only way to jointly provide cores, geophysical logs, subsurface fluid samples, and in situ stress measurements that are necessary to identify (1) key geochemical–mechanical processes that govern the evolution of volcanic rock properties and (2) the thermochemical–biological processes that facilitate subsurface mineral growth and dissolution. The varied rock textures and intense fluid circulations that likely changed through time make caldera–rift systems excellent localities to understand interactions between fluids (magmatic and meteoric) and different lithologies, both within and outside fault zones.
2.4 Topic 3: deep biosphere diversity, function, and geobiological interactions
Very little is known of the extent of deep subsurface biospheres, how they are sustained, how they respond to geologic processes (e.g. seismic or magmatic activity), and how they alter fluid or rock properties. This is due in large part to logistical difficulties in accessing suitable environments or samples for study and, more importantly, in coordinating sampling events with geologic events (e.g. earthquakes). Fluid build-up in volcanic environments can be released through seismic activity, with localised subsurface effects occurring in both local and linked, but distinct, geothermal systems (Payne et al., 2019). Further, low-magnitude seismic events may increase the connectivity of fluids in the subsurface, expose fresh mineral surfaces for water–rock interactions, and release trapped substrates or even generate new substrates (e.g. methane, hydrogen, hydrogen peroxide, or sulfate) capable of supporting microbial metabolisms (Telling et al., 2015; Stone et al., 2022). Finally, extensive fracturing and faults in caldera–rift systems permit fluid flow that can further concentrate volcanically derived volatiles (e.g. CO2 or H2S) (Lowenstern et al., 2015) that can support subsurface microbiomes.
Collectively, these observations offer a compelling argument for seismic and volcanic activity directly supporting subsurface microbial activity, although the characteristics, magnitudes, and timescales of such responses are only beginning to be studied (e.g. Payne et al., 2019). There is therefore a critical need to develop new approaches, techniques, and infrastructure to assess responses of subsurface biospheres to geologic processes, particularly in volcanically and seismically active regions. As of now, they are mainly investigated through their surface manifestations (hot springs), which indicate that subsurface microbial communities are acutely responsive and sensitive to spatial changes in the physicochemical composition of aquifers sourcing the springs (Colman et al., 2021; Fullerton et al., 2021; Power et al., 2023). Collectively, these studies are strong indicators that subsurface litho-autotrophic microbial ecosystems (SliMEs) in hydrothermal systems would similarly be responsive to input of geogenic electron donors or acceptors, regardless of the process involved (e.g. increased connectivity of aquifers, release of fluid inclusions, or increased water–rock interactions).
In addition to the influences of geophysical, geochemical, and hydrological processes on subsurface biospheres, microbial activities also exert feedback that can alter subsurface fluid, rock, and mineral properties. However, little is known of the extent of microbial influences on these properties, and particularly at temperatures greater than 40 °C (Magnabosco et al., 2018). Indirect evidence from surface features suggests that subsurface microbial communities alter hydrothermal fluid compositions through their metabolism of inorganic substrates, the acidification of hydrothermal waters (Mosser et al., 1973; Nordstrom et al., 2005; Colman et al., 2022), consumption of gases (e.g. H2 and CH4) (Wankel et al., 2011), alteration of minerals (Casar et al., 2020; Templeton and Caro, 2023), and precipitation of minerals (e.g. calcite) (Barry et al., 2019). The extent of these activities, their potential to significantly alter mineral assemblages, and their ability to predict geologic events remain poorly understood.
Scientific drilling is the only way to coordinate sampling subsurface fluids and the SliMEs they support to address the critical need to identify the temporal nature and indicators of functional responses with volcanic and tectonic events common to caldera systems. Borehole monitoring provides the required natural observatory to explore the feedbacks between microbial activity, the geosphere, and the hydrosphere. The diversity of magmatic and hydrothermal processes at the OVC implies a similar diversity of such ecosystems, both spatially and temporally, including in a borehole connected to fluids at varied temperature and composition.
2.5 Summary: scientific drilling in caldera–rift settings can best address global knowledge gaps
Calderas located in rifts are ideal natural laboratories for addressing CALDERA's research topics and global knowledge gaps. In caldera–rift systems, volcanic eruptions and earthquakes occur frequently, both separately and together, making them ideal environments for identifying and evaluating precursors of eruptions of varied sizes and styles. Large heat sources and faults result in dynamic fluid circulations that support a diverse biosphere.
Only scientific drilling can provide the subsurface, continuous, multi-disciplinary, and high-resolution records insulated from surface processes and furthermore provide later access for decadal monitoring. While there are thousands of boreholes for commercial geothermal energy use in terrestrial calderas, access to data from these boreholes is commonly limited by both technical constraints imposed by high temperatures (> 150 °C) and confidentiality. Groundwater wells are shallow (< 100 m) and often lack cores. Hot springs are often unsuitable analogues to the deep hydrothermal fluids and biosphere because they are subject to extensive mixing with near-surface fluids and are infused by oxidised atmospheric gases that exert strong influences on hot spring microbiology (Colman et al., 2019). Ocean scientific drillings in hydrothermal settings have investigated aspects such as seawater–rock interactions (Bach et al., 2003), but they remain sparse, cannot be directly transferred to low-salinity systems, and rarely involve in situ stress measurements or monitoring. The CALDERA programme is ideally positioned to address multiple knowledge gaps.
2.6 Lessons arising from CALDERA will be applicable globally
The CALDERA project proposes, for the first time, to develop understanding of the complete volcanic–tectonic–hydrological–biological system in a caldera–rift system. Mutually enriching relationships with communities, including Indigenous Māori, will advance good practice guidelines for such projects globally.
The new multi-disciplinary datasets and models developed at the OVC will unlock understanding at other volcanoes, calderas, rifts, and hydrothermal systems. The precursors to earthquakes and volcanic eruptions identified at the OVC will be transferable to caldera systems globally. Clarification of feedbacks between magmatic or meteoric fluid flow and faults will be generally applicable to improving understanding of crustal processes and assessing geohazards and the sustainable use of resources. Findings on volcanic rock properties and evolutionary alteration processes will significantly grow the global dataset. The OVC will serve as an unprecedented model system to quantify the influence of seismicity and volcanic unrest on the activity and function of microbial communities, resulting in a comprehensive understanding of global subsurface biosphere activities and responsiveness to associated environmental drivers.
By targeting moderate temperatures (40–150 °C) and groundwater–hydrothermal systems, CALDERA is complementary to other scientific drilling projects targeting deeper supercritical and magma systems (e.g. Newberry – NDDP, USA; Krafla – KMT and IDDP, Iceland; Japan – JBBP). With the clear expression of the Taupō Rift, CALDERA is complementary to the ICDP/IODP project at the partly submerged and weakly rifted Campi Flegrei caldera (Italy, De Natale et al., 2016). IODP expeditions to Brothers Volcano in the Kermadec Arc offshore New Zealand (Expedition 376, de Ronde et al., 2019) and to the Hellenic Arc Volcanic Field (Expedition 398, Druitt et al., 2022) will provide onshore–offshore comparisons of arc volcanisms, caldera processes, and reactions of ecosystems to volcanic eruptions.
In addition to increased scientific understanding of Earth processes occurring in rifted calderas, CALDERA will significantly contribute to improving societal goals.
3.1 Geohazards: increase resilience to volcanic and seismic hazards
A major caldera-forming eruption (volcanic explosivity index VEI > 6–7) would have severe human, economic, and climatic repercussions globally (Self, 2006). While smaller eruptions are less devastating than large ones, their higher frequency may pose the most significant risks to local and regional communities and can lead to national-scale social and economic disruption (e.g. the 1886 Tarawera eruption at the OVC; Rowe et al., 2021). Earthquakes that occur during volcanic eruptions (sometimes M > 6.0; Villamor et al., 2011) are difficult to include in seismic hazard models. Caldera unrest generates high interest and concern among local communities (e.g. during the 2022 Taupō caldera unrest, Aotearoa New Zealand). A global analysis by the European Science Foundation calculated the benefits of understanding and monitoring supervolcanoes at USD 0.5–3.5 billion per annum (Plag et al., 2015).
Converting scientific knowledge into tailored hazard planning advice allows decision-makers to better anticipate and assess the volcanic and seismic hazards through optimised monitoring and education programmes, leading to improved community resilience. This outcome is aligned with ICDP Theme 2 Geohazards and addresses key targets of the UNDRR – Sendai framework For Disaster Risk Reduction 2015–2030.
3.2 Georesources: foster the sustainable use and protection of hydrothermal and groundwater resources
Large populations live near calderas and their geothermal resources (e.g. in Kenya, Mexico, and Japan; Lund et al., 2022). Communities also need access to freshwater for drinking, farming, and recreational uses. Technologies to extract metals (e.g. Au, Ag, or Li) and silica (Simmons et al., 2016) and to store CO2 are rapidly emerging in geothermal operations. Although geothermal energy is already produced from calderas worldwide, new or expanded developments are limited by high economic risk due to limitations in (1) mapping heat sources, fluid pathways and fluid chemistry as well as understanding their spatiotemporal variations (Jolie et al., 2021); (2) quantifying meteoric water recharge; and (3) understanding links between subsurface and surface water features that are important culturally, spiritually (especially for Indigenous communities), economically (e.g. bathing, cooking, and tourism), and generally for wellbeing.
Fostering the sustainable management, protection, and access to hydrothermal and groundwater resources aligns with ICDP Theme 3 Georesources, contributes to the UNESCO sustainable development goals, and supports reduction of CO2 emissions under the Paris Agreement.
3.3 Deep biosphere
Microorganisms and their metabolic activities drive Earth's biogeochemical cycles that support and sustain global ecosystem health (Falkowski et al., 2008). Geological processes in continental hydrothermal systems (fluid circulation inducing physicochemical variations, mineral precipitation or dissolution) are known to drive microbial community composition and activity (Fullerton et al., 2021), but less is known about the responses of microbial communities to rapid geological events like those in caldera–rift settings. The extent, rate, and sensitivity of these responses remain to be determined, constituting the next frontier of environmental microbiology studies.
Understanding how and when such substrates are available to subsurface microbiomes is central to understanding their role in Earth's biogeochemical cycles and generally the development of Earth's hydrosphere–atmosphere–biosphere system (ICDP Theme 1, Geodynamic processes). Moreover, a better-resolved understanding of subsurface biosphere–geosphere interactions in active volcanic regions would enable the development of microbial indicators of volcanic unrest (ICDP Theme 2, Geohazards). Finally, studies of little-understood subsurface microbiomes would improve strategies for conserving and managing these critical microbial ecosystems, improve estimates of greenhouse gas budgets, promote new biotechnological developments including renewable energy production and greenhouse gas storage (ICDP Theme 3, Georesources), and inform the search for life in rocky exoplanet subsurface settings.
4.1 Drilling plan
The CALDERA project aims to collect cores, geophysical logs, in situ stress measurements, and downhole fluid samples and to provide long-term (ca. 10-year) borehole access for monitoring. Borehole data will be interpreted in the context of local- and regional-scale surface datasets. To achieve the scientific and societal objectives, drilling must
-
be located in a zone of active seismicity to test the fluid and biosphere response to nearby earthquakes;
-
intersect volcanic products pre-dating the last caldera-forming eruption (Rotoiti Ignimbrite, ∼ 55–60 ka) that are likely missing or poorly represented from surface records;
-
collect cores, downhole logs, and fluid samples across an active permeable fault to reveal fault zone textures as well as mechanical and hydraulic properties;
-
measure the downhole pressure and temperature as boreholes warm up to locate all permeable intervals and measure the well injectivity and productivity;
-
collect fluid samples to quantify the mixing between meteoric recharge and deep magmatic–hydrothermal fluids, sample the subsurface biosphere, and evaluate their effects on mineral alterations;
-
be located near hot springs to establish potential hydraulic and biosphere connections between the subsurface and surface, including in response to seismic or volcanic activity;
-
intersect the water table at shallow depth and high-permeability rocks to allow for natural fluid recharge within a few days, i.e. a timescale relevant for measuring a microbial response;
-
obtain cores from rocks not too hydrothermally altered in at least some borehole sections to provide characteristics of eruptions and a complete chronostratigraphy (usually difficult for hydrothermally altered samples);
-
collect cores and geophysical logs across a variety of lithologies to maximise learning about volcanic rock properties and fluid–rock interactions together with their effects on fault properties, fluid circulations and chemistry, and biosphere composition and activity;
-
reach 1000–1500 m depth to limit near-surface effects on in situ stress measurements (topographic effects), fluids, rock properties, and fault splays; and
-
have at least parts of the boreholes at < 40–120 °C, where the biosphere is readily detectable and known to be active, and overall < 150 °C to be able to rely on conventional downhole tools: a gentle temperature gradient would be ideal for evaluating changes in biosphere, fluid, and rock properties as a function of temperature.
The precise drilling location, configuration, and activities will be decided based on these scientific requirements together with landowners, local Māori, and regulatory agencies. Lake beds and wahi tapu (sacred land) areas are excluded due to cultural sensitivity.
Social license will be carefully assessed during site surveys, drilling planning, and drilling and monitoring through community engagements, particularly regarding mātauranga and values. The CALDERA project does not seek to modify the volcanic environment, suppress natural processes, or interact directly with magma, which will facilitate gaining of community support. The scientific boreholes will be used for monitoring rather than the continuous fluid extraction or injection conducted in the numerous geothermal and groundwater boreholes in the TVZ. Public risk perception of a potential unforecasted volcanic or seismic event triggered by drilling (Cassidy et al., 2023) will be mitigated in several ways. First, extensive social engagement will communicate that the project aims to monitor rather than modify the volcano. Early communication about the volcano's behaviour prior to drilling, including potential periods of unrest (seismic swarms), will lower the likelihood that a period of unrest may be perceived as caused by drilling. Second, deliberately targeting the margins and outside parts of the caldera and staying away from magma (spatially and with depth) will decrease potential safety and ethical concerns. Third, the project will follow current regulatory processes that require community support, low environmental impact, and safety assessments. Obtaining regulatory permits will be facilitated by the ongoing discussions with regional councils and their attendance at the workshop. Hundreds of geothermal, mineral, and water drillings have been conducted in the TVZ since the 1950s, including at the nearby Kawerau and Rotorua geothermal fields. None of these wells has intersected magma and, due to the TVZ being a naturally seismic area, induced microseismicity has not caused public concern (Sherburn et al., 2015a, b). Finally, an evaluation and risk mitigation plan will be conducted prior to drilling, using experience gained from drilling TVZ geothermal fields, and in other ICDP projects (e.g. the DFDP-2 project targeting the Alpine Fault, Chamberlain et al., 2017; in preparation for magma drilling of the Krafla Magma Testbed, Ilic et al., 2021). Those plans will be supported by decades of experience in volcano and seismic monitoring and community engagement in the TVZ.
Three potential drilling areas were delineated (Fig. 2). They are located outside the caldera structural margin, so that stratigraphy is not dominated by thick intracaldera deposits of large caldera-forming eruptions that can exceed 1 km thickness (e.g. Rosenberg et al., 2020). Two locations (labelled “1” and “3” in Fig. 2) contain mapped active faults where past volcano-tectonic activity is documented (Berryman et al., 2022; Villamor et al., 2022), currently experience frequent seismic activity (Bannister et al., 2022), and have nearby hot springs. Products pre-dating the last caldera-forming eruption (55–60 ka) are shallow in Area 3, as abandoned geothermal wells to the south of the area intersected the Waiotapu Ignimbrite (0.71 ± 0.06 Ma) at 236 m (Grindley, 1963). In Area 1, the top of the Rotoiti Ignimbrite crops out in eroded valleys (Villamor et al., 2022). Area 2 (Puhipuhi Basin) contains a structural caldera margin clearly imaged by gravity and electric surveys (Lithgow et al., 2022; Miller et al., 2022b). Thick (potentially > 100 m) recent (post-Rotoiti Ignimbrite) pumice deposits prohibit paleoseismic studies. This area experienced a deep seismic swarm in 2019 (Benson et al., 2021). The Puhipuhi Basin is hydrothermally altered and contains possible mineralisation. The biosphere has been studied in multiple springs at Waimangu near Area 3 (Power et al., 2018).
Achieving the multi-disciplinary objectives in this complex system requires at least two boreholes. Excellent existing tephra records provide multiple marker layers for stratigraphic correlations. At least one borehole should be inclined (i.e. deviated from the vertical) to intersect the entirety of a fault and its damage zone, as fault dip angles are generally high in the TVZ (60–80°; Villamor et al., 2017b; McNamara et al., 2019). A shallow borehole (about 200 m depth, temperature < 120 °C) would primarily focus on biosphere and hydrology aspects, especially connections between the subsurface and surface. A deep borehole (1000–1500 m depth) would primarily focus on stratigraphy, tectonics, in situ stress, rock properties, and hydrothermal fluids, including monitoring of volcano-tectonic activity and changes in deep fluids. Biosphere studies would also be conducted in this deep borehole if temperatures permit. Site characterisation will ensure that stratigraphic and structural targets are reached, but estimating the temperature profile prior to drilling will be difficult. Indeed, convection dominates in the TVZ, and lateral or downward-directed groundwater flows commonly interact with ascending hydrothermal fluids.
Table 1Potential drilling configurations (illustrated in Fig. 4). Shallow means ∼ 200 m depth, and deep means ∼ 10 000–1500 m depth.
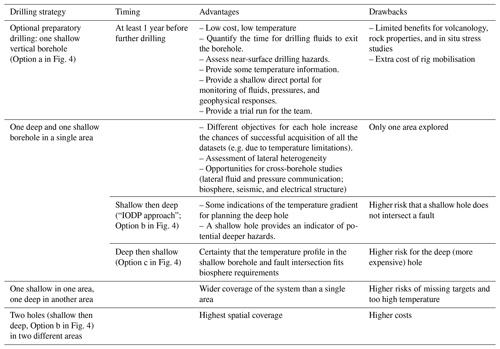
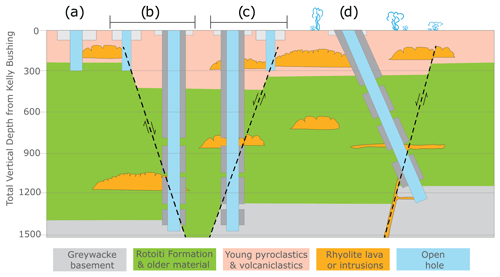
Figure 4Potential drilling scenarios (see Table 1). The geology is speculative, with the Rotoiti Formation (last caldera-forming eruption at ∼ 55–60 ka) and older deposits thickening towards the centre of the caldera, i.e. not necessarily with constant thickness across the faults. Lavas and especially intrusions (dikes and sills) are difficult to image prior to drilling. The cased hole sections (light and dark grey) represent near-surface “conductor” casing and deeper cemented borehole casing. Open-hole or perforated sections exist where no casing is shown. (a) Shallow preliminary test hole to ∼ 200 m depth. (b) Shallow then deep borehole. (c) Deep then shallow borehole. Deep holes in panels (b) and (c) could be either vertical or inclined to optimise steep fault intersection. An example of an inclined borehole is presented in panel (d).
Several drilling configurations were discussed during the workshop (Table 1, Fig. 4). Drilling a deep borehole and a shallow borehole in the same area increases the chances of addressing the multi-disciplinary objectives and provides opportunities for cross-borehole studies (e.g. tracer tests and variations in rock properties, fluids, and the biosphere) but restricts the exploration of the complex caldera system to a single area. If drilled in the same area, drilling a shallow borehole first (possibly as part of site characterisation) de-risks the deep, more expensive borehole. Drilling pairs of boreholes in two areas offers the best coverage of the OVC and maximises research outcomes but is more expensive. The final drilling configuration will be determined following detailed site surveys of the three target locations.
The modest temperature and depth targets facilitate the use of conventional drilling materials and a large suite of downhole logging measurements, downhole fluid sampling, installation of optic fibres for monitoring (e.g. distributed acoustic and temperature sensing – DAS, DTS), and other long-term observational instruments (e.g. seismometer, tiltmeter). Extended leak-off tests will be run at casing points for in situ stress measurements. Packers for permeability and in situ stress measurements will be deployed if the temperature is within operating limits. An online gas monitoring system (OLGA, Erzinger et al., 2006) during drilling and transient well tests will also be used.
Biosphere studies will focus on post-drilling downhole fluid sampling using the Kinetically Activated Subsurface Microbial Sampler (KASMS). The KASMS is designed to autonomously collect and preserve up to six sets of fluid samples for use in quantifying microbial cell number and activity, to conduct molecular analyses (i.e. to identify shifts in the taxonomic and functional composition and the diversity of microbial communities), and to conduct geochemical analyses. The KASMS can be triggered by a seismic tremor above a set minimum magnitude or otherwise triggered remotely on demand, which then initiates a series of sample collection processes at user-defined time intervals (Freifeld et al., 2005). The KASMS will be essential for evaluating the response of SliMEs to nearby earthquakes in hydrothermal systems. Problematic microbial contamination from drilling will be minimised by sampling only post-drilling. Therefore, UV filtering of drilling fluids will not be needed, which substantially simplifies drilling planning and reduces costs. The time needed for water-based drilling fluids to dissipate after drilling will need to be evaluated to avoid contamination.
4.2 Site characterisation
The extensive collection of the existing datasets at the OVC (see the Supplement) will (1) facilitate drill-site selection and (2) enable borehole samples to be placed in a regional-scale context. Based on gravity inversions, the depth of the basement top is estimated to be between 1000 and 2000 m depth in the three areas (Stagpoole et al., 2021; Miller et al., 2022b). Faults are well mapped onto a surface using lidar combined with paleoseismic trenches in Areas 1 and 3 (two and seven trenches, respectively, accompanied by ground-penetrating radar profiles across fault strands) (Berryman et al., 2008, 2022; Villamor et al., 2022). Magnetotelluric surveys at 2 km spacing cover Area 2 (Bertrand et al., 2022) and are scheduled to be acquired in Area 3 in the next few years. Airborne magnetic data cover all the areas.
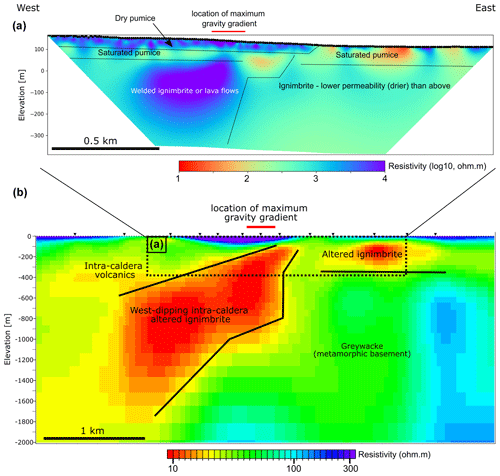
Figure 5Geophysical imaging of the eastern OVC caldera margin (location in Fig. 1). (a) Electrical resistivity tomography. (b) Magnetotelluric. The dotted square shows the extent of panel (a). The red bar indicates the maximum gravity gradient. The colour maps are optimised for the data ranges of each survey. After Lithgow et al. (2022).
An electrical resistivity tomography (ERT) line (2 km long, 10 m electrode spacing) and a 2-D magnetotelluric profile (4 km long, 13 sites) (Lithgow et al., 2022) were surveyed over the OVC's steepest gravity gradient in Area 2. Despite a lack of topographical relief and surface fault expression caused by infill by recent pumice material, the survey clearly imaged the eastern margin of the OVC (Fig. 5).
To further refine the drilling sites, we will undertake multiple geophysical surveys. We will deploy a dense nodal seismic array to image faults in the uppermost 1 km and potentially stratigraphic markers. Closely spaced gravity measurements will refine the depth to basement and basement fault offsets, especially in Areas 1 and 3, where the existing data are sparse. Drone magnetic surveys in areas with poorer airborne coverage will improve hydrothermal alteration mapping. A joint ERT and magnetotelluric profile in Areas 1 and 3 will refine fault location and identify possible hot (or saline) fluid or clay alteration. Self potential and CO2 soil gas surveys will help determine groundwater flow and connections to deep magmatic sources. A seismic reflection experiment with a 2-D profile of transit time tomography will be considered to locate faults, although the highly scattering subsurface produces poor imaging in the TVZ. Shallow fault imaging may also include additional paleoseismic trenching, ground-penetrating radar, and a shear wave land streamer system (Polom et al., 2016).
Shallow drill cuttings from the Kawerau Geothermal Field will be revisited to identify the base of the Rotoiti Ignimbrite. Spring temperature, chemistry, and isotopes in the OVC region will be compiled. If necessary, additional fluid sampling will be conducted for fluid and biosphere studies, based on geographical distribution and altitude, fault alignments, and surface geology, to provide a framework for interpreting downhole fluids and the biosphere.
4.3 Data and sample management plan
The OVC lies within the rohe (territories) of multiple iwi. As recognised by the 1840 Te Tiriti o Waitangi (Treaty of Waitangi) and the United Nations Declaration on the Rights of Indigenous Peoples (UNDRIP), this accords mana whenua authority over data and/or samples (geological, water, biological) collected from the OVC. Reflecting this, the data and sample management plan applied in this research programme will be guided by the principles of Indigenous data governance (Kukutai et al., 2023) and will be co-designed with mana whenua (e.g. as in Power et al., 2024). In parallel, the data and sample management plan will also need to reflect the funding agencies' requirements that increasingly require all data to be open access. For example, data generated during drilling operations (e.g. metadata from drilling, core and subsurface drilling, and borehole logs) will be stored on site in the ICDP mobile Drilling Information System (mDIS). Hence, we will apply both the F.A.I.R. (findable, accessible, interoperable, reusable) and C.A.R.E. (collective benefit, authority to control, responsibility, and ethics) guiding principles (Wilkinson et al., 2016; Carroll et al., 2020) for scientific data management and Indigenous data governance. This will also provide a proof of concept for future international drilling programmes.
The CALDERA workshop supported by the ICDP refined the scientific goals of the project idea and confirmed that scientific drilling is required to address these globally important science and societal goals. The OVC is an excellent setting to conduct the project, and we have articulated how findings at the OVC would be applied elsewhere. The workshop attendees reinforced the importance of studying the volcanic, tectonic, hydrological, and biosphere processes in connection rather than in isolation. Unravelling the changes through time and particularly the response of the system to volcanic or tectonic activity was deemed of high interest. It was agreed that the multi-disciplinary objectives and the natural complexity of the system require at least two boreholes: one shallow (< 200 m) focused on biosphere and groundwater, and one deep (1000–1500 m) focused on volcanic stratigraphy, tectonics, hydrology, and physical rock properties. Long-term monitoring provides opportunities to study the caldera–rift system in between and during times of volcanic or seismic activity. The workshop also introduced the participants to Māori world views and interests that are integral to the CALDERA project.
Karakia Whakawātea
Kia tau ngā manaakitanga a Te Mea Ngaro
Ki runga i tēnā, i tēnā o tātau
Kia mahea ai te hua mākihikihi
Kia toi te kupu, toi te mana, toi te whenua
Tūturu whakamaua kia tīna. Tīna!
Haumi e, Hui e, Tāiki e.
Bestow the blessings of the unseen force
upon each and everyone
Clear our path of any obstructions
And the words, the prestige and the land flourish
Indeed let it be affirmed!
Let it be binding, together, all in agreement
No codes or datasets were used in this article.
The supplement related to this article is available online at: https://doi.org/10.5194/sd-33-67-2024-supplement.
Ludmila Adam (School of Environment, University of Auckland, Auckland, New Zealand), Hiroshi Asanuma (AIST, Fukushima, Japan), Jeff Ashby (Webster Drilling, Porirua, New Zealand), Jennifer Biddle (School of Marine Science and Policy, University of Delaware, Lewes, USA), Eric S. Boyd (Department of Microbiology and Cell Biology, Montana State University, Bozeman, USA), Andrea Brogi (Department of Earth and Geo-environmental Sciences, University of Bari Aldo Moro, Bari, Italy), S. Craig Cary (Thermophile Research Unit, University of Waikato, Hamilton, New Zealand), Corentin Caudron (Département Géosciences Environnement Société, Université Libre de Bruxelles, Bruxelles, Belgium), Daniel R. Colman (Department of Microbiology and Cell Biology, Montana State University, Bozeman, USA), Alysia Cox (Department of Chemistry & Geochemistry, Montana Technological University, Butte, USA), Penny Doorman (Bay Of Plenty Regional Council, Whakatāne, New Zealand), Jennifer Eccles (School of Environment, University of Auckland, Auckland, New Zealand), Mark Gibson (Department of Commercial and Business Partnerships, GNS Science, Taupō, New Zealand), Guido Giordano (University of Roma, Italy), Gino Gonzales (University of Bari Aldo Moro, Bari, Italy), Zayre Yvonne González Acevedo (Geology Department, Centro de Investigación Científica y de Educación Superior de Ensenada, Baja California, Ensenada, Mexico), Luke Goodwin (Webster Drilling, Porirua, New Zealand), Magnus Tumi Gudmundsson (Institute of Earth Sciences, School of Engineering and Natural Sciences, University of Iceland, Reykjavik, Iceland), John Hadfield (Waikato Regional Council, Hamilton, New Zealand), Kim Handley (Department of Biological Sciences, University of Auckland, Auckland, New Zealand), Ery Hughes (Department of Earth Structure and Processes, GNS Science, Lower Hutt, New Zealand), Finnigan Illsley-Kemp (School of Geography, Environment and Earth Sciences, Victoria University of Wellington, Wellington, New Zealand), Mark Ivamy (Bay Of Plenty Regional Council, Rotorua, New Zealand), Marie Jackson (Department of Geology and Geophysics, University of Utah, Salt Lake City, USA), Franziska Keller (Institute for Geochemistry and Petrology, ETH, Zurich, Switzerland), Geoff Kilgour (Department of Earth Structure and Processes, GNS Science, Taupō, New Zealand), Matteo Lelli (Institute of Geosciences and Earth Resources, IGG-CNR, Pisa Italy), Domenico Liotta (Department of Earth and Geo-environmental Sciences, University of Bari Aldo Moro, Bari; NRC, Pisa, Italy), Haibo Liu (College of Earth Sciences, Jilin University, Jilin, China), Karen G. Lloyd (Department of Microbiology, University of Tennessee, Knoxville, USA), Katherine Luketina (Waikato Regional Council, Hamilton, New Zealand), Tiipene Marr (Te Mana o Ngāti Rangitihi, Whakatāne, New Zealand), Cécile Massiot (Department of Earth Resources and Materials, GNS Science, Lower Hutt, New Zealand), David D. McNamara (Department of Earth, Ocean and Ecological Sciences, University of Liverpool, Liverpool, UK), Sarah D. Milicich (Department of Earth Resources and Materials, GNS Science, Lower Hutt, New Zealand), Craig A. Miller (Department of Earth Structure and Processes, GNS Science, Taupō, New Zealand), Santanu Misra (Department of Earth Sciences, IITK, Uttar Pradesh, India), Giordano Montegrossi (Istituto di Geoscienze e Georisorse, IGG-CNR, Firenze, Italy), Madison Myers (Department of Earth Sciences, Montana State University, Bozeman, USA), Alexander R. L. Nichols (School of Earth and Environment, University of Canterbury, Christchurch, New Zealand), German Orozco (Department of Earth Resources and Materials, GNS Science, Taupō, New Zealand), Simona Pierdominici (Department of Geomechanics and Scientific Drilling, GFZ, Potsdam, Germany), Shane M. Rooyakkers (Department of Earth Resources and Materials, GNS Science, Lower Hutt, New Zealand), Monika Rusiecka (Institut des Sciences de la Terre, Université d'Orléans, Orléans, France), Anja Schleicher (Department of Inorganic and Isotope Geochemistry, GFZ, Potsdam, Germany), Douglas R. Schmitt (Department of Earth, Atmospheric, and Planetary Sciences, Purdue University, West Lafayette, USA), Brad Scott (Department of Earth Structure and Processes, GNS Science, Taupō, New Zealand), Andri Stefansson (School of Engineering and Natural Science, University of Iceland, Reykjavik, Iceland), John Stix (Department of Earth & Planetary Sciences, McGill University, Montreal, Canada), Matthew B. Stott (School of Biological Sciences, University of Canterbury, Christchurch, New Zealand), Camille Thomas (Université de Genève, Genève; Institute of Geological Sciences and Oeschger Centre for Climate Research, University of Bern, Switzerland), Kingan Tony (Webster Drilling, Porirua, New Zealand), John Townend (School of Geography, Environment and Earth Sciences, Victoria University of Wellington, Wellington, New Zealand), Pri Utami (Department of Geological Engineering, Gadjah Mada University, Yogyakarta, Indonesia), Kasper van Wijk (Department of Physics, University of Auckland, Auckland, New Zealand), Pilar Villamor (Department of Earth Structure and Processes, GNS Science, Lower Hutt, New Zealand), Pujun Wang (College of Earth Sciences, Jilin University, Jilin, China), Bain Webster (Webster Drilling, Porirua, New Zealand), Paul White (Department of Surface Geosciences, GNS Science, Taupō, New Zealand), Thomas Wiersberg (GFZ, Potsdam, Germany), Ralph Winmill (Contact Energy, Taupō, New Zealand), Zhuolong Yang (College of Earth Sciences, Jilin University, Jilin, China), Sadiq J. Zarrouk (Department of Engineering Science, University of Auckland, Auckland, New Zealand), Christian Zeeden (Department of petrophysics and borehole geophysics, Leibniz Institute for Applied Geophysics, Hannover, Germany), and Mariana Zuquim (Bay Of Plenty Regional Council, Whakatāne, New Zealand).
CM, LA, SCC, EH, GK, TM, SDM, CAM, ARLN, SMR, MBS, PV, and SJZ convened the workshop in Tauranga. All the co-authors contributed to the text and figures that resulted in the submission of a proposal. SMR, PV, EH, CAM, and JS focused on hypothesis 1; LA, DRS, ML, DL, AS, DDM, and SDM on hypothesis 2; and MBS, DRC, CT, ESB, SCC, and AC on hypothesis 3. EH compiled the Supplement. CM, CAM, CT, PV, and SP led the revisions, editing, and homogenisation of the final version of the paper. Participants at the workshop contributed intellectual input.
The contact author has declared that none of the authors has any competing interests.
Publisher’s note: Copernicus Publications remains neutral with regard to jurisdictional claims made in the text, published maps, institutional affiliations, or any other geographical representation in this paper. While Copernicus Publications makes every effort to include appropriate place names, the final responsibility lies with the authors.
Thanks to Ulrich Harms and the rest of the ICDP-OSG for advice on preparation of the workshop. We thank Tamati Waaka for the Karakia Whakawātea and all the iwi representatives who contributed to early discussions about the project. We acknowledge all the participants for their constructive input and enthusiasm. Thanks to Tomo Morishita for editorial handling, Mattia Pitstone for reviews, and an anonymous reviewer for their comments that helped improve the manuscript. Those interested in participating in future CALDERA activities are invited to contact the authors at caldera.drill@gns.cri.nz.
The authors of this paper wish to acknowledge with deep sadness the passing of one of our co-authors, S. Craig Cary. His enthusiasm and extensive expertise significantly contributed to the CALDERA project development since its early days, including for the ICDP workshop and this paper. He will be dearly missed.
The workshop was largely funded by the International Continental Scientific Drilling Program, with additional support from Tourism New Zealand, Geodiscovery New Zealand, and the University of Waikato. The CALDERA project development was partially funded by New Zealand government funds through GNS Science's Strategic Science Investment Fund programme “Understanding the Te Riu-a-Māui/Zealandia Continent”. A Toka Tū Ake EQC Biennial Grant supports a site survey and the associated community engagement.
This paper was edited by Tomoaki Morishita and reviewed by Mattia Pistone and one anonymous referee.
Abe, K.: Seismicity of the caldera-making eruption of Mount Katmai, Alaska in 1912, B. Seismol. Soc. Am., 82, 175–191, https://pubs.geoscienceworld.org/ssa/bssa/article/82/1/175/119528/Seismicity-of-the-caldera-making-eruption-of-Mount (last access: 18 March 2024), 1992.
Acocella, V.: Structural control on magmatism along divergent and convergent plate boundaries: Overview, model, problems, Earth Sci. Rev., 136, 226–288, https://doi.org/10.1016/j.earscirev.2014.05.006, 2014.
Acocella, V., Neri, M., Behncke, B., Bonforte, A., Del Negro, C., and Ganci, G.: Why Does a Mature Volcano Need New Vents? The Case of the New Southeast Crater at Etna, Front. Earth Sci., 4, 67, https://doi.org/10.3389/feart.2016.00067, 2016.
Allan, A. S. R., Wilson, C. J. N., Millet, M.-A., and Wysoczanski, R. J.: The invisible hand: Tectonic triggering and modulation of a rhyolitic supereruption, Geology, 40, 563–566, https://doi.org/10.1130/G32969.1, 2012.
Arnórsson, S.: Geothermal systems in Iceland: Structure and conceptual models – I. High-temperature areas, Geothermics, 24, 561–602, https://doi.org/10.1016/0375-6505(95)00025-9, 1995.
Bach, W., Peucker-Ehrenbrink, B., Hart, S. R., and Blusztajn, J. S.: Geochemistry of hydrothermally altered oceanic crust: DSDP/ODP Hole 504B – Implications for seawater-crust exchange budgets and Sr- and Pb-isotopic evolution of the mantle, Geochem. Geophy. Geosy., 4, 8904, https://doi.org/10.1029/2002GC000419, 2003.
Bannister, S., Bertrand, E. A., Heimann, S., Bourguignon, S., Asher, C., Shanks, J., and Harvison, A.: Imaging sub-caldera structure with local seismicity, Okataina Volcanic Centre, Taupo Volcanic Zone, using double-difference seismic tomography, J. Volcanol. Geoth. Res., 431, 107653, https://doi.org/10.1016/j.jvolgeores.2022.107653, 2022.
Barker, S. J., Rowe, M. C., Wilson, C. J. N., Gamble, J. A., Rooyakkers, S. M., Wysoczanski, R. J., Illsley-Kemp, F., and Kenworthy, C. C.: What lies beneath? Reconstructing the primitive magmas fueling voluminous silicic volcanism using olivine-hosted melt inclusions, Geology, 48, 504–508, https://doi.org/10.1130/G47422.1, 2020.
Barry, P. H., de Moor, J. M., Giovannelli, D., Schrenk, M., Hummer, D. R., Lopez, T., Pratt, C. A., Segura, Y. A., Battaglia, A., Beaudry, P., Bini, G., Cascante, M., d'Errico, G., di Carlo, M., Fattorini, D., Fullerton, K., Gazel, E., González, G., Halldórsson, S. A., Iacovino, K., Ilanko, T., Kulongoski, J. T., Manini, E., Martínez, M., Miller, H., Nakagawa, M., Ono, S., Patwardhan, S., Ramírez, C. J., Regoli, F., Smedile, F., Turner, S., Vetriani, C., Yücel, M., Ballentine, C. J., Fischer, T. P., Hilton, D. R., and Lloyd, K. G.: Forearc carbon sink reduces long-term volatile recycling into the mantle, Nature, 568, 487–492, https://doi.org/10.1038/s41586-019-1131-5, 2019.
Battaglia, M., Troise, C., Obrizzo, F., Pingue, F., and De Natale, G.: Evidence for fluid migration as the source of deformation at Campi Flegrei caldera (Italy), Geophys. Res. Lett., 33, L01307, https://doi.org/10.1029/2005GL024904, 2006.
Benson, T. W., Illsley-Kemp, F., Elms, H. C., Hamling, I. J., Savage, M. K., Wilson, C. J. N., Mestel, E. R. H., and Barker, S. J.: Earthquake Analysis Suggests Dyke Intrusion in 2019 Near Tarawera Volcano, New Zealand, Front. Earth Sci., 8, 606992, https://doi.org/10.3389/feart.2020.606992, 2021.
Berryman, K., Villamor, P., Nairn, I., van Dissen, R., Begg, J., and Lee, J.: Late Pleistocene surface rupture history of the Paeroa Fault, Taupo Rift, New Zealand, New Zeal. J. Geol. Geop., 51, 135–158, https://doi.org/10.1080/00288300809509855, 2008.
Berryman, K., Villamor, P., Nairn, I., Begg, J., Alloway, B. V, Rowland, J., Lee, J., and Capote, R.: Volcano-tectonic interactions at the southern margin of the Okataina Volcanic Centre, Taupō Volcanic Zone, New Zealand, J. Volcanol. Geoth. Res., 427, 107552, https://doi.org/10.1016/j.jvolgeores.2022.107552, 2022.
Bertrand, E. A., Kannberg, P., Caldwell, T. G., Heise, W., Constable, S., Scott, B., Bannister, S., Kilgour, G., Bennie, S. L., Hart, R., and Palmer, N.: Inferring the magmatic roots of volcano-geothermal systems in the Rotorua Caldera and Okataina Volcanic Centre from magnetotelluric models, J. Volcanol. Geoth. Res., 431, 107645, https://doi.org/10.1016/j.jvolgeores.2022.107645, 2022.
Bouvet de Maisonneuve, C., Forni, F., and Bachmann, O.: Magma reservoir evolution during the build up to and recovery from caldera-forming eruptions – A generalizable model?, Earth Sci. Rev., 218, 103684, https://doi.org/10.1016/j.earscirev.2021.103684, 2021.
Bursik, M., Renshaw, C., McCalpin, J., and Berry, M.: A volcanotectonic cascade: Activation of range front faulting and eruptions by dike intrusion, Mono Basin-Long Valley Caldera, California, J. Geophys. Res.-Sol. Ea., 108, 2393, https://doi.org/10.1029/2002JB002032, 2003.
Byerlee, J. D. and Savage, J. C.: Coulomb plasticity within the fault zone, Geophys. Res. Lett., 19, 2341–2344, https://doi.org/10.1029/92GL02370, 1992.
Cabaniss, H. E., Gregg, P. M., and Grosfils, E. B.: The Role of Tectonic Stress in Triggering Large Silicic Caldera Eruptions, Geophys. Res. Lett., 45, 3889–3895, https://doi.org/10.1029/2018GL077393, 2018.
Carroll, S. R., Garba, I., Figueroa-Rodríguez, O. L., Holbrook, J., Lovett, R., Materechera, S., Parsons, M., Raseroka, K., Rodriguez-Lonebear, D., and Rowe, R.: The CARE principles for indigenous data governance, Data Sci. J., 19, 43, https://doi.org/10.5334/dsj-2020-043, 2020.
Carson, L. B., Miller, C. A., Massiot, C., Villamor, P., Leonard, G. S., and Alcaraz, S. A.: 3D visualisation model of the basement geology and caldera structure at Okataina Volcanic Centre, in: Geoscience Society of New Zealand Annual Conference, 29 November–1 December 2022, Massey University, Palmerston North, New Zealand, edited by: Zernack, A. V. and Palmer, J., Geoscience Society of New Zealand, Wellington, NZ, 161A, ISBN 978-0-473-66216-5, 2022.
Casar, C. P., Kruger, B. R., Flynn, T. M., Masterson, A. L., Momper, L. M., and Osburn, M. R.: Mineral-hosted biofilm communities in the continental deep subsurface, Deep Mine Microbial Observatory, SD, USA, Geobiology, 18, 508–522, https://doi.org/10.1111/gbi.12391, 2020.
Cassidy, M., Sandberg, A., and Mani, L.: The Ethics of Volcano Geoengineering, Earths Future, 11, 1–17, https://doi.org/10.1029/2023EF003714, 2023.
Chamberlain, C. J., Boese, C. M., Eccles, J. D., Savage, M. K., Baratin, L. M., Townend, J., Gulley, A. K., Jacobs, K. M., Benson, A., Taylor-Offord, S., and Thurber, C.: Real-time earthquake monitoring during the second phase of the deep fault drilling project, Alpine Fault, New Zealand, Seismol. Res. Lett., 88, 1443–1454, https://doi.org/10.1785/0220170095, 2017.
Cluff, M. A., Hartsock, A., MacRae, J. D., Carter, K., and Mouser, P. J.: Temporal Changes in Microbial Ecology and Geochemistry in Produced Water from Hydraulically Fractured Marcellus Shale Gas Wells, Environ. Sci. Technol., 48, 6508–6517, https://doi.org/10.1021/es501173p, 2014.
Cole, J. W., Deering, C. D., Burt, R. M., Sewell, S., Shane, P. A. R., and Matthews, N. E.: Okataina Volcanic Centre, Taupo Volcanic Zone, New Zealand: A review of volcanism and synchronous pluton development in an active, dominantly silicic caldera system, Earth Sci. Rev., 128, 1–17, https://doi.org/10.1016/j.earscirev.2013.10.008, 2014.
Colman, D. R., Lindsay, M. R., and Boyd, E. S.: Mixing of meteoric and geothermal fluids supports hyperdiverse chemosynthetic hydrothermal communities, Nat. Commun., 10, 681, https://doi.org/10.1038/s41467-019-08499-1, 2019.
Colman, D. R., Lindsay, M. R., Harnish, A., Bilbrey, E. M., Amenabar, M. J., Selensky, M. J., Fecteau, K. M., Debes II, R. V, Stott, M. B., Shock, E. L., and Boyd, E. S.: Seasonal hydrologic and geologic forcing drive hot spring geochemistry and microbial biodiversity, Environ. Microbiol., 23, 4034–4053, https://doi.org/10.1111/1462-2920.15617, 2021.
Colman, D. R., Amenabar, M. J., Fernandes-Martins, M. C., and Boyd, E. S.: Subsurface Archaea associated with rapid geobiological change in a model Yellowstone hot spring, Commun. Earth. Environ., 3, 205, https://doi.org/10.1038/s43247-022-00542-2, 2022.
Corti, G.: Evolution and characteristics of continental rifting: Analog modeling-inspired view and comparison with examples from the East African Rift System, Tectonophysics, 522–523, 1–33, https://doi.org/10.1016/j.tecto.2011.06.010, 2012.
Costa, F.: Clocks in magmatic rocks, Annu. Rev. Earth. Planet Sci., 49, 231–252, https://doi.org/10.1146/annurev-earth-080320-060708, 2021.
De Natale, G., Troise, C., Mark D, Mormone, A., Piochi, M., Di Vito, M. A., Isaia, R., Carlino, S., Barra, D., and Somma, R.: The Campi Flegrei Deep DrillingProject (CFDDP): New insight on caldera structure, evolution and hazard implications for the Naples area (Southern Italy), Geochem. Geophy. Geosy., 17, 4836–4847, https://doi.org/10.1002/2015GC006183, 2016.
de Ronde, C. E. J., Humphris, S. E., Höfig, T. W., and Reyes, A. G.: Critical role of caldera collapse in the formation of seafloor mineralization: The case of Brothers volcano, Geology, 47, 762–766, https://doi.org/10.1130/G46047.1, 2019.
Druitt, T., Kutterolf, S., and Höfig, T. W.: Expedition 398 Scientific Prospectus: Hellenic Arc Volcanic Field, International Ocean Discovery Program, https://doi.org/10.14379/iodp.sp.398.2022, 2022.
Ellis, S., Heise, W., Kissling, W., Villamor, P., and Schreurs, G.: The effect of crustal melt on rift dynamics: case study of the Taupo Volcanic Zone, New Zeal. J. Geol. Geop., 57, 453–458, https://doi.org/10.1080/00288306.2014.972961, 2014.
Erzinger, J., Wiersberg, T., and Zimmer, M.: Real-time mud gas logging and sampling during drilling, Geofluid, 6, 225–233, https://doi.org/10.1111/j.1468-8123.2006.00152.x, 2006.
Falkowski, P. G., Fenchel, T., and Delong, E. F.: The Microbial Engines That Drive Earth's Biogeochemical Cycles, Science, 320, 1034–1039, https://doi.org/10.1126/science.1153213, 2008.
Fournier, R. O.: Hydrothermal processes related to movement of fluid from plastic into brittle rock in the magmatic-epithermal environment, Econ. Geol., 94, 1193–1211, https://doi.org/10.2113/gsecongeo.94.8.1193, 1999.
Freifeld, B. M., Trautz, R. C., Kharaka, Y. K., Phelps, T. J., Myer, L. R., Hovorka, S. D., and Collins, D. J.: The U-tube: A novel system for acquiring borehole fluid samples from a deep geologic CO2 sequestration experiment, J. Geophys. Res.-Sol. Ea., 110, B10203, https://doi.org/10.1029/2005JB003735, 2005.
Fullerton, K. M., Schrenk, M. O., Yücel, M., Manini, E., Basili, M., Rogers, T. J., Fattorini, D., Di Carlo, M., d'Errico, G., Regoli, F., Nakagawa, M., Vetriani, C., Smedile, F., Ramírez, C., Miller, H., Morrison, S. M., Buongiorno, J., Jessen, G. L., Steen, A. D., Martínez, M., de Moor, J. M., Barry, P. H., Giovannelli, D., and Lloyd, K. G.: Effect of tectonic processes on biosphere–geosphere feedbacks across a convergent margin, Nat. Geosci., 14, 301–306, https://doi.org/10.1038/s41561-021-00725-0, 2021.
Giggenbach, W. F.: Variations in the chemical and isotopic composition of fluids discharged from the Taupo Volcanic Zone, New Zealand, J. Volcanol. Geoth. Res., 68, 89–116, https://doi.org/10.1016/0377-0273(95)00009-J, 1995.
Gold, T.: The deep, hot biosphere, P. Natl. Acad. Sci. USA, 89, 6045–6049, https://doi.org/10.1073/pnas.89.13.6045, 1992.
Gottsmann, J., Carniel, R., Coppo, N., Wooller, L., Hautmann, S., and Rymer, H.: Oscillations in hydrothermal systems as a source of periodic unrest at caldera volcanoes: Multiparameter insights from Nisyros, Greece, Geophys. Res. Lett., 34, L07307, https://doi.org/10.1029/2007GL029594, 2007.
Grindley, G. W.: Geology and structure of Waiotapu geothermal field, New Zealand Department of Scientific and Industrial Research bulletin, 155, 10–25, 1963.
Hayba, D. O. and Ingebritsen, S. E.: Multiphase groundwater flow near cooling plutons, J. Geophys. Res.-Sol. Ea., 102, 12235–12252, https://doi.org/10.1029/97JB00552, 1997.
Heap, M. J. and Violay, M. E. S.: The mechanical behaviour and failure modes of volcanic rocks: a review, B. Volcanol., 83, 33, https://doi.org/10.1007/s00445-021-01447-2, 2021.
Heap, M. J., Troll, V. R., Kushnir, A. R. L., Gilg, H. A., Collinson, A. S. D., Deegan, F. M., Darmawan, H., Seraphine, N., Neuberg, J., and Walter, T. R.: Hydrothermal alteration of andesitic lava domes can lead to explosive volcanic behaviour, Nat. Commun., 10, 5063, https://doi.org/10.1038/s41467-019-13102-8, 2019.
Hikuroa, D.: Mātauranga Māori – the ūkaipō of knowledge in New Zealand, J. Roy. Soc. New Zeal., 47, 5–10, https://doi.org/10.1080/03036758.2016.1252407, 2017.
Hildreth, W.: The timing of caldera collapse at Mount Katmai in response to magma withdrawal toward Novarupta, Geophys. Res. Lett., 18, 1541–1544, https://doi.org/10.1029/91GL01083, 1991.
Hill, D. P., Pollitz, F., and Newhall, C.: Earthquake–volcano interactions, Phys. Today, 55, 41–47, https://doi.org/10.1063/1.1535006, 2002.
Hilley, G. E., Brodsky, E.E., Roman, D., Shillington, D. J., Brudzinski, M., Behn, M., Tobin, H., and the SZ4D RCN: SZ4D Implementation Plan, edited by: Hilley, G. E., Stanford Digital Repository, https://doi.org/10.25740/hy589fc7561, 2022.
Houghton, B. F., Wilson, C. J. N., McWilliams, M. O., Lanphere, M. A., Weaver, S. D., Briggs, R. M., and Pringle, M. S.: Chronology and dynamics of a large silicic magmatic system: Central Taupo Volcanic Zone, New Zealand, Geology, 23, 13–16, https://doi.org/10.1130/0091-7613(1995)023<0013:CADOAL>2.3.CO;2, 1995.
Hughes, E. C., Mazot, A., Kilgour, G., Asher, C., Michelini, M., Britten, K., Chardot, L., Feisel, Y., and Werner, C.: Understanding degassing pathways along the 1886 Tarawera (New Zealand) volcanic fissure by combining soil and lake CO2 fluxes, Front. Earth Sci., 7, 264, https://doi.org/10.3389/feart.2019.00264, 2019.
Hughes, G. R. and Mahood, G. A.: Silicic calderas in arc settings: Characteristics, distribution, and tectonic controls, Bulletin, 123, 1577–1595, https://doi.org/10.1130/B30232.1, 2011.
Hutnak, M., Hurwitz, S., Ingebritsen, S. E., and Hsieh, P. A.: Numerical models of caldera deformation: Effects of multiphase and multicomponent hydrothermal fluid flow, J. Geophys. Res.-Sol. Ea., 114, B04411, https://doi.org/10.1029/2008JB006151, 2009.
Ilic, O., Sigmundsson, F., Lavallée, Y., Mortensen, A. K., Eichelberger, J., Markusson, S. H., Papale, P., and Thordarson, T.: Geological Risk Associated with Drilling into Magma at Krafla Caldera, Iceland: Preliminary Evaluation, in: Proceedings of the World Geothermal Congress 2020+1, Reykjavik, Iceland and online, April–October 2021, International Geothermal Association, https://www.geothermal-energy.org/pdf/IGAstandard/WGC/2020/12145.pdf (last access: 18 March 2024), 2021.
Illsley-Kemp, F., Barker, S. J., Wilson, C. J. N., Chamberlain, C. J., Hreinsdóttir, S., Ellis, S., Hamling, I. J., Savage, M. K., Mestel, E. R. H., and Wadsworth, F. B.: Volcanic Unrest at Taupō Volcano in 2019: Causes, Mechanisms and Implications, Geochem., Geophy. Geosy. 22, e2021GC009803, https://doi.org/10.1029/2021GC009803, 2021.
Jolie, E., Klinkmueller, M., Moeck, I., and Bruhn, D.: Linking gas fluxes at Earth's surface with fracture zones in an active geothermal field, Geology, 44, 187–190, https://doi.org/10.1130/G37412.1, 2016.
Jolie, E., Scott, S., Faulds, J., Chambefort, I., Axelsson, G., Gutiérrez-Negrín, L. C., Regenspurg, S., Ziegler, M., Ayling, B., and Richter, A.: Geological controls on geothermal resources for power generation, Nat. Rev. Earth Environ., 2, 324–339, https://doi.org/10.1038/s43017-021-00154-y, 2021.
Kanakiya, S., Turner, G. M., Rowe, M. C., Adam, L., and Lindsay, J. M.: High remanent magnetization measured in hydrothermally altered lavas, Geophys. Res. Lett., 48, e2021GL095732, https://doi.org/10.1029/2021GL095732, 2021a.
Kanakiya, S., Adam, L., Rowe, M. C., Lindsay, J. M., and Esteban, L.: The role of tuffs in sealing volcanic conduits, Geophys. Res. Lett., 48, e2021GL095175, https://doi.org/10.1029/2021GL095175, 2021b.
Kennedy, B. M., Farquhar, A., Hilderman, R., Villeneuve, M. C., Heap, M. J., Mordensky, S., Kilgour, G., Jolly, Art., Christenson, B., and Reuschlé, T.: Pressure Controlled Permeability in a Conduit Filled with Fractured Hydrothermal Breccia Reconstructed from Ballistics from Whakaari (White Island), New Zealand, Geosciences, 10, 138, https://doi.org/10.3390/geosciences10040138, 2020.
Komori, S., Kagiyama, T., Takakura, S., Ohsawa, S., Mimura, M., and Mogi, T.: Effect of the hydrothermal alteration on the surface conductivity of rock matrix: comparative study between relatively-high and low temperature hydrothermal systems, J. Volcanol. Geoth. Res., 264, 164–171, https://doi.org/10.1016/j.jvolgeores.2013.08.009, 2013.
Kukutai, T., Campbell-Kamariera, K., Mead, A., Mikaere, K., Moses, C., Whitehead, J., and Cormack, D.: Māori data governance model, Te Kāhui Raraunga, https://tengira.waikato.ac.nz/__data/assets/pdf_file/0008/973763/Maori_Data_Governance_Model.pdf (last access: 18 March 2024), 2023.
Lahitte, P., Gillot, P.-Y., and Courtillot, V.: Silicic central volcanoes as precursors to rift propagation: the Afar case, Earth Planet Sci. Lett., 207, 103–116, https://doi.org/10.1016/S0012-821X(02)01130-5, 2003.
Lazrus, H., Maldonado, J., Blanchard, P., Souza, M. K., Thomas, B., and Wildcat, D.: Culture change to address climate change: Collaborations with Indigenous and Earth sciences for more just, equitable, and sustainable responses to our climate crisis, PLOS Climate, 1, e0000005, https://doi.org/10.1371/journal.pclm.0000005, 2022.
Linde, A. T. and Sacks, I. S.: Triggering of volcanic eruptions, Nature, 395, 888–890, https://doi.org/10.1038/27650, 1998.
Lithgow, R., Massiot, C., Bertrand, E., Heise, W., Miller, C. A., Bennie, S., Brakenrig, T., Coup, L., and Macdonald, N.: Interpretation of geophysical data from the eastern margin of the Okataina Volcanic Centre (Issue October), GNS Science, Lower Hutt, NZ, https://doi.org/10.21420/FPEK-CG53, 2022.
Lowenstern, J. B., Smith, R. B., and Hill, D. P.: Monitoring super-volcanoes: geophysical and geochemical signals at Yellowstone and other large caldera systems, Philos. t. roy. soc. A, 364, 2055–2072, https://doi.org/10.1098/rsta.2006.1813, 2006.
Lowenstern, J. B., Bergfeld, D., Evans, W. C., and Hunt, A. G.: Origins of geothermal gases at Yellowstone, J. Volcanol. Geoth. Res., 302, 87–101, https://doi.org/10.1016/j.jvolgeores.2015.06.010, 2015.
Lund, J. W., Huttrer, G. W., and Toth, A. N.: Characteristics and trends in geothermal development and use, 1995 to 2020, Geothermics, 105, 102522, https://doi.org/10.1016/j.geothermics.2022.102522, 2022.
Magnabosco, C., Lin, L. H., Dong, H., Bomberg, M., Ghiorse, W., Stan-Lotter, H., Pedersen, K., Kieft, T. L., van Heerden, E., and Onstott, T. C.: The biomass and biodiversity of the continental subsurface, Nat. Geosci., 11, 707–717, https://doi.org/10.1038/s41561-018-0221-6, 2018.
Mahony, S. H., Wallace, L. M., Miyoshi, M., Villamor, P., Sparks, R. S. J., and Hasenaka, T.: Volcano-tectonic interactions during rapid plate-boundary evolution in the Kyushu region, SW Japan, Bulletin, 123, 2201–2223, https://doi.org/10.1130/B30408.1, 2011.
Marzocchi, W.: Remote seismic influence on large explosive eruptions, J. Geophys. Res.-Sol. Ea., 107, EPM 6-1–EPM 6-7, https://doi.org/10.1029/2001JB000307, 2002.
Mazot, A., Schwandner, F. M., Christenson, B., De Ronde, C. E., Inguaggiato, S., Scott, B., Graham, D., Britten, K., Keeman, J., and Tan, K.: CO2 discharge from the bottom of volcanic Lake Rotomahana, New Zealand, Geochem. Geophy. Geosy., 15, 577–588, https://doi.org/10.1002/2013GC004945, 2014.
McNamara, D. D., Lister, A., and Prior, D. J.: Calcite sealing in a fractured geothermal reservoir: Insights from combined EBSD and chemistry mapping, J. Volcanol. Geoth. Res., 323, 38–52, https://doi.org/10.1016/j.jvolgeores.2016.04.042, 2016.
McNamara, D. D., Milicich, S. D., Massiot, C., Villamor, P., McLean, K., Sépulveda, F., and Ries, W. F.: Tectonic Controls on Taupo Volcanic Zone Geothermal Expression: Insights From Te Mihi, Wairakei Geothermal Field, Tectonics, 38, 3011–3033, https://doi.org/10.1029/2018TC005296, 2019.
Miller, C. A. and Jolly, A. D.: A model for developing best practice volcano monitoring: A combined threat assessment, consultation and network effectiveness approach, Nat. Hazards, 71, 493–522, https://doi.org/10.1007/s11069-013-0928-z, 2014.
Miller, C. A., Taylor-Offord, S., Sherburn, S., Magill, C. R., and Pastor-Paz, J.: Revised threat assessment of New Zealand's volcanoes, GNS Science, Lower Hutt (NZ), GNS Science report; 2022/41, 32 pp., https://doi.org/10.21420/AQWM-ZR12, 2022a.
Miller, C. A., Barretto, J., Stagpoole, V., Caratori-Tontini, F., Brakenrig, T., and Bertrand, E.: The integrated history of repeated caldera formation and infill at the Okataina Volcanic Centre: Insights from 3D gravity and magnetic models, J. Volcanol. Geoth. Res., 427, 107555, https://doi.org/10.1016/j.jvolgeores.2022.107555, 2022b.
Mordensky, S. P., Villeneuve, M. C., Farquharson, J. I., Kennedy, B. M., Heap, M. J., and Gravley, D. M.: Rock mass properties and edifice strength data from Pinnacle Ridge, Mt. Ruapehu, New Zealand, J. Volcanol. Geoth. Res., 367, 46–62, https://doi.org/10.1016/j.jvolgeores.2018.09.012, 2018.
Mordensky, S. P., Heap, M. J., Kennedy, B. M., Gilg, H. A., Villeneuve, M. C., Farquharson, J. I., and Gravley, D. M.: Influence of alteration on the mechanical behaviour and failure mode of andesite: implications for shallow seismicity and volcano monitoring, B. Volcanol., 81, 1–12, https://doi.org/10.1007/s00445-019-1306-9, 2019.
Morgavi, D., Arienzo, I., Montagna, C., Perugini, D., and Dingwell, D. B.: Magma mixing: history and dynamics of an eruption trigger, in: Volcanic Unrest, edited by: Gottsmann, J., Neuberg, J., and Scheu, B., Springer Open, 123–137, https://doi.org/10.1007/978-3-319-58412-6, 2017.
Mosser, J. L., Mosser, A. G., and Brock, T. D.: Bacterial origin of sulfuric acid in geothermal habitats, Science, 179, 1323–1324, https://doi.org/10.1126/science.179.4080.1323, 1973.
Mu, A. and Moreau, J. W.: The geomicrobiology of CO2 geosequestration: a focused review on prokaryotic community responses to field-scale CO2 injection, Front. Microbiol., 6, 263, https://doi.org/10.3389/fmicb.2015.00263, 2015.
Muirhead, J. D., Illsley-Kemp, F., Barker, S. J., Villamor, P., Wilson, C. J. N., Otway, P., Mestel, E. R. H., Leonard, G. S., Ellis, S., Savage, M. K., Bannister, S., Rowland, J. V, Townsend, D., Hamling, I. J., Hreinsdóttir, S., Smith, B., McGregor, R., Snowden, M., and Shalla, Y.: Stretching, Shaking, Inflating: Volcanic-Tectonic Interactions at a Rifting Silicic Caldera, Front. Earth Sci., 10, 835841, https://doi.org/10.3389/feart.2022.835841, 2022.
Nairn, I. A.: Geology of the Okataina Volcanic Centre, scale 1 : 50 000, Institute of Geological and Nuclear Sciences Limited, ISBN 0478097182, 2002.
New Zealand Active Faults Database: 2003–, GNS Science, Lower Hutt (NZ), http://gns.cri.nz/Home/Products/Databases/Active-Faults-Database-of-New-Zealand (last access: 22 September 2023), 2003.
Nicolas, A., Lévy, L., Sissmann, O., Li, Z., Fortin, J., Gibert, B., and Sigmundsson, F.: Influence of hydrothermal alteration on the elastic behaviour and failure of heat-treated andesite from Guadeloupe, Geophys. J. Int., 223, 2038–2053, https://doi.org/10.1093/gji/ggaa437, 2020.
Nordstrom, D. K., Ball, J. W., and McCleskey, R. B.: Ground water to surface water: chemistry of thermal outflows in Yellowstone National Park, Geothermal biology and geochemistry in Yellowstone National Park, Open File Report, Montana State University, Bozeman, Montana, USA, 73–94, https://pubs.usgs.gov/publication/70198852 (last access: 18 March 2024), 2005.
Norini, G., Carrasco-Núñez, G., Corbo-Camargo, F., Lermo, J., Rojas, J. H., Castro, C., Bonini, M., Montanari, D., Corti, G., Moratti, G., Piccardi, L., Chavez, G., Zuluaga, M. C., Ramirez, M., and Cedillo, F.: The structural architecture of the Los Humeros volcanic complex and geothermal field, J. Volcanol. Geoth. Res., 381, 312–329, https://doi.org/10.1016/j.jvolgeores.2019.06.010, 2019.
Oliva, S. J., Ebinger, C. J., Wauthier, C., Muirhead, J. D., Roecker, S. W., Rivalta, E., and Heimann, S.: Insights into fault-magma interactions in an early-stage continental rift from source mechanisms and correlated volcano-tectonic earthquakes, Geophys. Res. Lett., 46, 2065–2074, https://doi.org/10.1029/2018GL080866, 2019.
Payne, D., Dunham, E. C., Mohr, E., Miller, I., Arnold, A., Erickson, R., Fones, E. M., Lindsay, M. R., Colman, D. R., and Boyd, E. S.: Geologic legacy spanning > 90 years explains unique Yellowstone hot spring geochemistry and biodiversity, Environ. Microbiol., 21, 4180–4195, https://doi.org/10.1111/1462-2920.14775, 2019.
Pearson-Grant, S., Miller, C. A., Carson, L. B., Bertrand, E. A., and Leonard, G. S.: Influences on geothermal circulation in the Okataina Volcanic Centre, New Zealand, J. Volcanol. Geoth. Res., 432, 107705, https://doi.org/10.1016/j.jvolgeores.2022.107705, 2022.
Plag, H. P., Brocklebank, S., Brosnan, D., Campus, P., Cloetingh, S., Jules-Plag, S., and Stein, S.: Extreme Geohazards: Reducing Disaster Risk and Increasing Resilience, European Science Fundation, 72 pp., ISBN 978-2-36873-197-0, http://archives.esf.org/fileadmin/Public_documents/Publications/Natural_Hazards.pdf (last access: 18 March 2024), 2015.
Pola, A., Crosta, G. B., Fusi, N., and Castellanza, R.: General characterization of the mechanical behaviour of different volcanic rocks with respect to alteration, Eng. Geol., 169, 1–13, https://doi.org/10.1016/j.enggeo.2013.11.011, 2014.
Polom, U., Mueller, C., Nicol, A., Villamor, P., Langridge, R. M., and Begg, J. G.: Finding the Concealed Section of the Whakatane Fault in the Whakatane Township with a Shear Wave Land Streamer System: A Seismic Surveying Report, Institute of Geological & Nuclear Sciences, ISBN 978-0-947510-76-3, 2016.
Power, J. F., Carere, C. R., Lee, C. K., Wakerley, G. L. J., Evans, D. W., Button, M., White, D., Climo, M. D., Hinze, A. M., and Morgan, X. C.: Microbial biogeography of 925 geothermal springs in New Zealand, Nat. Commun., 9, 2876, https://doi.org/10.1038/s41467-018-05020-y, 2018.
Power, J. F., Lowe, C. L., Carere, C. R., McDonald, I. R., Cary, S. C., and Stott, M. B.: Temporal dynamics of geothermal microbial communities in Aotearoa-New Zealand, Front. Microbiol., 14, 1094311, https://doi.org/10.3389/fmicb.2023.1094311, 2023.
Power, J. F., Carere, C. R., Welford, H. E., Hudson, D. T., Lee, K. C., Moreau, J. W., Ettema, T. J., Reysenbach, A. L., Lee, C. K., Colman, D. R., and Boyd, E. S.: A genus in the bacterial phylum Aquificota appears to be endemic to Aotearoa-New Zealand, Nat. Commun., 15, 179, https://doi.org/10.1038/s41467-023-43960-2, 2024.
Rosenberg, M. D., Wilson, C. J. N., Bignall, G., Ireland, T. R., Sepulveda, F., and Charlier, B. L. A.: Structure and evolution of the Wairakei–Tauhara geothermal system (Taupo Volcanic Zone, New Zealand) revisited with a new zircon geochronology, J. Volcanol. Geoth. Res., 390, 106705, https://doi.org/10.1016/j.jvolgeores.2019.106705, 2020.
Rowe, M. C., Carey, R. J., White, J. D. L., Kilgour, G., Hughes, E., Ellis, B., Rosseel, J.-B., and Segovia, A.: Tarawera 1886: an integrated review of volcanological and geochemical characteristics of a complex basaltic eruption, New Zeal. J. Geol. Geop., 64, 296–319, https://doi.org/10.1080/00288306.2021.1914118, 2021.
Rowland, J. V. and Simmons, S. F.: Hydrologic, magmatic, and tectonic controls on hydrothermal flow, Taupo Volcanic Zone, New Zealand: Implications for the formation of epithermal vein deposits, Econ. Geol., 107, 427–457, https://doi.org/10.2113/econgeo.107.3.427, 2012.
Rowland, J. V., Wilson, C. J. N., and Gravley, D. M.: Spatial and temporal variations in magma-assisted rifting, Taupo Volcanic Zone, New Zealand, J. Volcanol. Geoth. Res., 190, 89–108, https://doi.org/10.1016/j.jvolgeores.2009.05.004, 2010.
Ruz-Ginouves, J., Gerbault, M., Cembrano, J., Iturrieta, P., Leiva, F. S., Novoa, C., and Hassani, R.: The interplay of a fault zone and a volcanic reservoir from 3D elasto-plastic models: Rheological conditions for mutual trigger based on a field case from the Andean Southern Volcanic Zone, J. Volcanol. Geoth. Res., 418, 107317, https://doi.org/10.1016/j.jvolgeores.2021.107317, 2021.
Sanderson, D. J. and Nixon, C. W.: The use of topology in fracture network characterization, J. Struct. Geol., 72, 55–66, https://doi.org/10.1016/j.jsg.2015.01.005, 2015.
Scarpa, R., Bianco, F., Capuano, P., Castellano, M., D'Auria, L., Di Lieto, B., and Romano, P.: Historic unrest of the Campi Flegrei Caldera, Italy, in: Campi Flegrei: A Restless Caldera in a Densely Populated Area, edited by: Orsi, G., D'Antonio, M., and Civetta, L., Springer, Berlin, Heidelberg, 257–282, https://doi.org/10.1007/978-3-642-37060-1_10, 2022.
Scott, S., Driesner, T., and Weis, P.: Geologic controls on supercritical geothermal resources above magmatic intrusions, Nat. Commun., 6, 7837, https://doi.org/10.1038/ncomms8837, 2015.
Self, S.: The effects and consequences of very large explosive volcanic eruptions, Philos. T. Roy. Soc. A, 364, 2073–2097, https://doi.org/10.1098/rsta.2006.1814, 2006.
Shapiro, N. M., Droznin, D. V, Droznina, S. Y., Senyukov, S. L., Gusev, A. A., and Gordeev, E. I.: Deep and shallow long-period volcanic seismicity linked by fluid-pressure transfer, Nat. Geosci., 10, 442–445, https://doi.org/10.1038/ngeo2952, 2017.
Sherburn, S., Bromley, C., Bannister, S., Sewell, S. M., and Bourguignon, S.: New Zealand Geothermal Induced Seismicity: an overview, in: Proceedings World Geothermal Congress 2015, Melbourne, Australia, 19–25 April 2015, edited by: Horne, R. and Boyd, T., International Geothermal Association, 24009, 9 pp., https://pangea.stanford.edu/ERE/db/WGC/papers/WGC/2015/24009.pdf (last access: 18 March 2024), 2015a.
Sherburn, S., Sewell, S. M., Bourguignon, S., Cumming, W., Bannister, S., Bardsley, C., Winick, J., Quinao, J., and Wallis, I. C.: Microseismicity at Rotokawa geothermal field, New Zealand, 2008—2012, Geothermics, 54, 23–34, https://doi.org/10.1016/j.geothermics.2014.11.001, 2015b.
Sibson, R. H.: Fluid involvement in normal faulting, J. Geodyn., 29, 469–499, https://doi.org/10.1016/S0264-3707(99)00042-3, 2000.
Simmons, S. F., Brown, K. L., Browne, P. R. L., and Rowland, J. V.: Gold and silver resources in Taupo Volcanic Zone geothermal systems, Geothermics, 59, 205–214, https://doi.org/10.1016/j.geothermics.2015.07.009, 2016.
Simpson, M. P. and Bignall, G.: Undeveloped high-enthalpy geothermal fields of the Taupo Volcanic Zone, New Zealand, Geothermics, 59, 325–346, https://doi.org/10.1016/j.geothermics.2015.08.006, 2016.
Somr, M., Žák, J., Kabele, P., and Tomek, F.: Analysis of fracturing processes leading to caldera collapse, Earth Sci. Rev., 241, 104413, https://doi.org/10.1016/j.earscirev.2023.104413, 2023.
Sparks, R. S. J.: Forecasting volcanic eruptions, Earth Planet. Sci. Lett., 210, 1–15, https://doi.org/10.1016/S0012-821X(03)00124-9, 2003.
Sparks, R. S. J., Blundy, J. D., Cashman, K. V., Jackson, M., Rust, A., and Wilson, C. J. N.: Large silicic magma bodies and very large magnitude explosive eruptions, B. Volcanol., 84, 4–9, https://doi.org/10.1007/s00445-021-01510-y, 2022.
Stagpoole, V., Miller, C., Caratori Tontini, F., Brakenrig, T., and Macdonald, N.: A two million-year history of rifting and caldera volcanism imprinted in new gravity anomaly compilation of the Taupō Volcanic Zone, New Zealand, New Zeal. J. Geol. Geop., 64, 358–371, https://doi.org/10.1080/00288306.2020.1848882, 2021.
Stein, R. S.: The role of stress transfer in earthquake occurrence, Nature, 402, 605–609, https://doi.org/10.1038/45144, 1999.
Stone, J., Edgar, J. O., Gould, J. A., and Telling, J.: Tectonically-driven oxidant production in the hot biosphere, Nat. Commun., 13, 4529, https://doi.org/10.1038/s41467-022-32129-y, 2022.
Taute, N., Morgan, K., Ingham, J., Archer, R., and Fa'aui, T.: Māori values in geothermal management and development, AlterNative, 18, 548–555, https://doi.org/10.1177/11771801221118629, 2022.
Telling, J., Boyd, E. S., Bone, N., Jones, E. L., Tranter, M., MacFarlane, J. W., Martin, P. G., Wadham, J. L., Lamarche-Gagnon, G., Skidmore, M. L., Hamilton, T. L., Hill, E., Jackson, M., and Hodgson, D. A.: Rock comminution as a source of hydrogen for subglacial ecosystems, Nat. Geosci., 8, 851–855, https://doi.org/10.1038/ngeo2533, 2015.
Templeton, A. S. and Caro, T. A.: The Rock-Hosted Biosphere, Annu. Rev. Earth Planet. Sci., 51, 493–519, https://doi.org/10.1146/annurev-earth-031920-081957, 2023.
Uno, M., Koyanagawa, K., Kasahara, H., Okamoto, A., and Tsuchiya, N.: Volatile-consuming reactions fracture rocks and self-accelerate fluid flow in the lithosphere, P. Natl. Acad. Sci. USA, 119, e2110776118, https://doi.org/10.1073/pnas.2110776118, 2022.
van Wyk de Vries, B. and Merle, O.: The effect of volcanic constructs on rift fault patterns, Geology, 24, 643–646, https://doi.org/10.1130/0091-7613(1996)024<0643:TEOVCO>2.3.CO;2, 1996.
Villamor, B. P., Litchfield, N. J., Gómez-Ortiz, D., Martin-González, F., Alloway, B. V, Berryman, K. R., Clark, K. J., Ries, W. F., Howell, A., and Ansell, I. A.: Fault ruptures triggered by large rhyolitic eruptions at the boundary between tectonic and magmatic rift segments: The Manawahe Fault, Taupō Rift, New Zealand, J. Volcanol. Geoth. Res., 427, 107478, https://doi.org/10.1016/j.jvolgeores.2022.107478, 2022.
Villamor, P., Berryman, K. R., Nairn, I. A., Wilson, K., Litchfield, N., and Ries, W.: Associations between volcanic eruptions from Okataina volcanic center and surface rupture of nearby active faults, Taupo rift, New Zealand: Insights into the nature of volcano-tectonic interactions, GSA Bull., 123, 1383–1405, https://doi.org/10.1130/B30184.1, 2011.
Villamor, P., Berryman, K. R., Ellis, S. M., Schreurs, G., Wallace, L. M., Leonard, G. S., Langridge, R. M., and Ries, W. F.: Rapid Evolution of Subduction-Related Continental Intraarc Rifts: The Taupo Rift, New Zealand, Tectonics, 36, 2250–2272, https://doi.org/10.1002/2017TC004715, 2017a.
Villamor, P., Nicol, A., Seebeck, H., Townsend, D., Massiot, C., McNamara, D., Ries, W., Rowland, J., Milicich, S., and Alcaraz, S.: Tectonic structure and permeability in the Taupō rift: New insights from analysis of LIDAR derived DEMs, in: Proceedings 39th New Zealand Geothermal Workshop, 22–24 November 2017, Rotorua, New Zealand, University of Auckland, New Zealand, https://www.geothermal-energy.org/pdf/IGAstandard/NZGW/2017/025_Villamor-Final_.pdf (last access: 18 March 2024), 2017b.
Walter, T. R. and Amelung, F.: Volcanic eruptions following M ≥ 9 megathrust earthquakes: Implications for the Sumatra-Andaman volcanoes, Geology, 35, 539–542, https://doi.org/10.1130/G23429A.1, 2007.
Wankel, S. D., Germanovich, L. N., Lilley, M. D., Genc, G., DiPerna, C. J., Bradley, A. S., Olson, E. J., and Girguis, P. R.: Influence of subsurface biosphere on geochemical fluxes from diffuse hydrothermal fluids, Nat. Geosci., 4, 461–468, https://doi.org/10.1038/ngeo1183, 2011.
Wilkinson, M. D., Dumontier, M., Aalbersberg, I. J., Appleton, G., Axton, M., Baak, A., Blomberg, N., Boiten, J.-W., da Silva Santos, L. B., Bourne, P. E., Bouwman, J., Brookes, A. J., Clark, T., Crosas, M., Dillo, I., Dumon, O., Edmunds, S., Evelo, C. T., Finkers, R., Gonzalez-Beltran, A., Gray, A. J. G., Groth, P., Goble, C., Grethe, J. S., Heringa, J., 't Hoen, P. A. C., Hooft, R., Kuhn, T., Kok, R., Kok, J., Lusher, S. J., Martone, M. E., Mons, A., Packer, A. L., Persson, B., Rocca-Serra, P., Roos, M., van Schaik, R., Sansone, S.-A., Schultes, E., Sengstag, T., Slater, T., Strawn, G., Swertz, M. A., Thompson, M., van der Lei, J., van Mulligen, E., Velterop, J., Waagmeester, A., Wittenburg, P., Wolstencroft, K., Zhao, J., and Mons, B.: The FAIR Guiding Principles for scientific data management and stewardship, Sci. Data, 3, 160018, https://doi.org/10.1038/sdata.2016.18, 2016.
Wilson, C. J. N. and Rowland, J. V.: The volcanic, magmatic and tectonic setting of the Taupo Volcanic Zone, New Zealand, reviewed from a geothermal perspective, Geothermics, 59, 168–187, https://doi.org/10.1016/j.geothermics.2015.06.013, 2016.
Wilson, C. J. N., Houghton, B. F., McWilliams, M. O., Lanphere, M. A., Weaver, S. D., and Briggs, R. M.: Volcanic and structural evolution of Taupo Volcanic Zone, New Zealand: a review, J. Volcanol. Geoth. Res., 68, 1–28, https://doi.org/10.1016/0377-0273(95)00006-G, 1995.
Wilson, C. J., Gravley, D. M., Leonard, G. S., and Rowland, J. V.: Volcanism in the central Taupo Volcanic Zone, New Zealand: tempo, styles and controls, Studies in volcanology: the legacy of George Walker, Special Publications of IAVCEI, 2, 225–247, 2009.
Wilson, C. J. N., Cooper, G. F., Chamberlain, K. J., Barker, S. J., Myers, M. L., Illsley-Kemp, F., and Farrell, J.: No single model for supersized eruptions and their magma bodies, Nat. Rev. Earth Environ., 2, 610–627, https://doi.org/10.1038/s43017-021-00191-7, 2021.
Wyering, L. D., Villeneuve, M. C., Wallis, I. C., Siratovich, P., Kennedy, B. M., Gravley, D. M., and Cant, J. L.: Mechanical and physical properties of hydrothermally altered rocks, Taupo Volcanic Zone, New Zealand, J. Volcanol. Geoth. Res., 288, 76–93, https://doi.org/10.1016/j.jvolgeores.2014.10.008, 2014.
Yang, T. H. J., Chambefort, I., Mazot, A., Rowe, M. C., Scott, B., Macdonald, N., Werner, C., Fischer, T. P., and de Ronde, C. E.: Understanding caldera degassing from a detailed investigation at Lake Rotoiti, Okataina Volcanic Centre, New Zealand, J. Volcanol. Geoth. Res., 433, 107716, https://doi.org/10.1016/j.jvolgeores.2022.107716, 2023.
Zhan, Y. and Gregg, P. M.: How accurately can we model magma reservoir failure with uncertainties in host rock rheology?. J. Geophys. Res.-Sol. Ea., 124, 8030–8042, https://doi.org/10.1029/2019JB018178, 2019.
Zucchi, M., Brogi, A., Liotta, D., Rimondi, V., Ruggieri, G., Montegrossi, G., Caggianelli, A., and Dini, A.: Permeability and hydraulic conductivity of faulted micaschist in the eastern Elba Island exhumed geothermal system (Tyrrhenian sea, Italy): insights from Cala Stagnone, Geothermics, 70, 125–145, https://doi.org/10.1016/j.geothermics.2017.05.007, 2017.
- Abstract
- Introduction
- Global research needs in active caldera–rift systems: CALDERA science plan
- Societal relevance
- Proposed strategies for scientific drilling
- Conclusion
- Code and data availability
- Team list
- Author contributions
- Competing interests
- Disclaimer
- Acknowledgements
- Financial support
- Review statement
- References
- Supplement
- Abstract
- Introduction
- Global research needs in active caldera–rift systems: CALDERA science plan
- Societal relevance
- Proposed strategies for scientific drilling
- Conclusion
- Code and data availability
- Team list
- Author contributions
- Competing interests
- Disclaimer
- Acknowledgements
- Financial support
- Review statement
- References
- Supplement