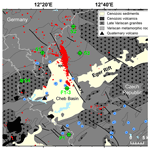
ICDP drilling of the Eger Rift observatory: magmatic fluids driving the earthquake swarms and deep biosphere
Tomáš Fischer
Torsten Dahm
Heiko Woith
Tomáš Vylita
Matthias Ohrnberger
Josef Vlček
Josef Horálek
Petr Dědeček
Martin Zimmer
Martin P. Lipus
Simona Pierdominici
Jens Kallmeyer
Frank Krüger
Katrin Hannemann
Michael Korn
Horst Kämpf
Thomas Reinsch
Jakub Klicpera
Daniel Vollmer
Kyriaki Daskalopoulou
The new in situ geodynamic laboratory established in the framework of the ICDP Eger project aims to develop the most modern, comprehensive, multiparameter laboratory at depth for studying earthquake swarms, crustal fluid flow, mantle-derived CO2 and helium degassing, and processes of the deep biosphere. In order to reach a new level of high-frequency, near-source and multiparameter observation of earthquake swarms and related phenomena, such a laboratory comprises a set of shallow boreholes with high-frequency 3-D seismic arrays as well as modern continuous real-time fluid monitoring at depth and the study of the deep biosphere.
This laboratory is located in the western part of the Eger Rift at the border of the Czech Republic and Germany (in the West Bohemia–Vogtland geodynamic region) and comprises a set of five boreholes around the seismoactive zone. To date, all monitoring boreholes have been drilled. This includes the seismic monitoring boreholes S1, S2 and S3 in the crystalline units north and east of the major Nový Kostel seismogenic zone, borehole F3 in the Hartoušov mofette field and borehole S4 in the newly discovered Bažina maar near Libá. Supplementary borehole P1 is being prepared in the Neualbenreuth maar for paleoclimate and biological research. At each of these sites, a borehole broadband seismometer will be installed, and sites S1, S2 and S3 will also host a 3-D seismic array composed of a vertical geophone chain and surface seismic array. Seismic instrumenting has been completed in the S1 borehole and is in preparation in the remaining four monitoring boreholes. The continuous fluid monitoring site of Hartoušov includes three boreholes, F1, F2 and F3, and a pilot monitoring phase is underway. The laboratory also enables one to analyze microbial activity at CO2 mofettes and maar structures in the context of changes in habitats. The drillings into the maar volcanoes contribute to a better understanding of the Quaternary paleoclimate and volcanic activity.
- Article
(12044 KB) - Full-text XML
- BibTeX
- EndNote
What are the physical and chemical processes leading to earthquake activity and fluid mobility? What are the pathways of fluids through the crust and how are they influenced by tectonic stress variations? How can geological processes influence the deep biosphere and the evolution of early life at depth? These are the open questions tackled by the ICDP project aimed at drilling in the Eger Rift region.
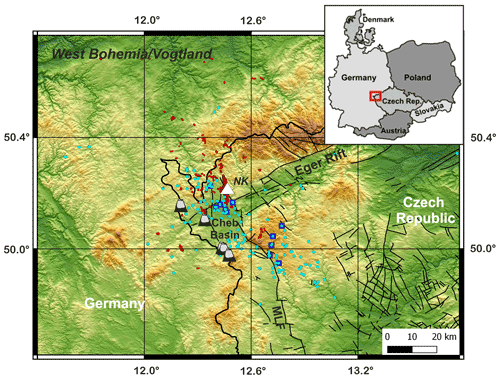
Figure 1The West Bohemia–Vogtland geodynamic region in the westernmost part of the Eger Rift: the epicenters of earthquakes from 1991 to 2021 are marked by red circles; cyan circles represent CO2 degassing; blue squares represent mofettes; the white triangle marks the Nový Kostel (NK) focal zone; the positions of five Quaternary volcanoes are indicated.
The western Eger Rift, cutting the Bohemian Massif in the westernmost part of the Czech Republic and the adjacent area in Germany, represents the West Bohemia–Vogtland region, which is one of the most unique European intracontinental geodynamic areas (Fig. 1). The geodynamic activity is represented by active magmatic underplating, mid-crustal earthquake swarms and massive diffuse degassing of mantle-derived CO2 (e.g., Bräuer et al., 2003; Horálek and Fischer, 2008). The region is also characterized by numerous mineral springs, mofettes, Tertiary–Quaternary volcanism, and neotectonic crustal movements located at the intersection of major intraplate fault and tectonic zones around the Cheb Basin. The close proximity and intensity of the different geodynamic processes in an intracontinental massif far from plate boundaries are outstanding, and it is likely that all of these phenomena are related to a common driver in the lithospheric upper mantle. Currently, it is well accepted that many earthquake swarms are driven by fluids in the crust. Nowadays, fluid migration and its control by tectonics are recognized in many regions worldwide under different tectonic and volcanic settings. The ICDP Eger Rift project provides extraordinary observations that help to unravel the relations between magmatic underplating, trans-crustal CO2 flux, earthquake swarms and their relation to volcanism and microbiology.
The evolution of the Eger Rift, a 300 km long, 50 km wide, ENE–WSW-trending zone and an active element of the European Cenozoic Rift System (Prodehl et al., 1995), is connected with the post-orogenic extension and the alkaline magmatic activity during the Cenozoic. The Cheb Basin developed at its southwestern end and dips to ∼ 300 m depth towards the east, where it is delimited by the escarpment of the Mariánské Lázně Fault. The basement of the Cheb Basin and its surroundings include the crystalline schists of the Saxothuringian unit and the granitoids of the Smrčiny Pluton, whereas the sedimentary fill of the Cheb Basin consists of Paleogene to Quaternary sediments (Kvaček and Teodoridis, 2007). Quaternary intraplate alkaline volcanism is documented at the western flank of the Cheb Basin in small volcanoes dated to 0.78–0.12 Ma (Mrlina et al., 2007; Wagner et al., 2002), whereas the CO2-dominated hydrothermal activity is dated to 0.23–0 Ma (Vylita et al., 2007). They comprise two Quaternary scoria cones (Železná hůrka/Eisenbühl and Komorní hůrka/Kammerbühl) and two volcanic maars (Mýtina and Neualbenreuth), where the eruptions are associated with phreatomagmatic and phreato-Strombolian activity (Geissler et al., 2005; Mrlina et al., 2009; Flechsig et al., 2015; Rohrmüller et al., 2017; Lied et al., 2020). The crustal structure of the area is complex with a wide zone of increased reflectivity at the crust–mantle transition (e.g., Tomek et al., 1997; Hrubcová et al., 2005, 2013; Hrubcová and Geissler, 2009). These lower crustal features may be interpreted as low-angle shear zones partly filled with fluids and/or small magmatic intrusions or partial melting as confirmed by mantle xenoliths (Geissler et al., 2005). In the upper crust, very distinct and highly reflective features (“bright spots”) were identified (Klemt, 2013; Schimschal, 2013; Mullick et al., 2015; Hrubcová et al., 2016), which can be related to the spatial–temporal behavior of seismic swarm activity and its fluid-driven origin.
The seismicity in the area is characterized by earthquake swarms, intensive long-lasting and low-magnitude seismicity, which contrasts with more typical mainshock–aftershock sequences. Such seismicity can be felt by the population and sometimes causes damage to buildings. It has been recognized in different areas worldwide, such as volcanic and geothermal fields (e.g., Dahm and Brandsdottir, 1997; Wyss et al., 1997; Lees, 1998; Dreger et al., 2000) and at the margins of tectonic plates (e.g., Einarsson, 1991), and can occur as a precursor to larger earthquakes, such as during the recent L'Aquila 2009 earthquake in Italy. However, the controlling processes and possible consequences of this kind of seismicity are still not fully disclosed.
In the western Eger Rift, the highest concentration of earthquake activity and CO2 degassing occurs in the area of the Cheb Basin, at the intersection of major tectonic lines with four Quaternary volcanoes. The ENE–WSW-trending Eger Graben and the N–S-trending earthquake zone between Vogtland and Leipzig (Grünthal et al., 2019), the Cheb–Domažlice Graben, and the morphologically expressed Mariánské Lázně Fault intersect at one location close to the main seismically active Nový Kostel (NK) zone (Fig. 1). It seems that the earthquake activity is related to the reactivation of a complex system of faults and tectonic zones, triggered by the ascent of magmatic fluids caused by ongoing magmatic process (Fischer et al., 2014; Bräuer et al., 2014) in which fluid channels to depth (CO2 conduits) play an important role (Kämpf et al., 2019). However, the understanding of magmatic activity, fluid ascent and earthquake-stimulating processes as well as their interconnections are not clear.
Fluid degassing of CO2 in the Cheb Basin and surrounding areas is in the form of wet and dry mofettes and mineral springs. High portions of mantle-derived helium and CO2 indicate a magmatic origin and fluid transport from the depleted lithospheric mantle (Weinlich et al., 1999; Bräuer et al., 2009), possibly related to active magmatic underplating (Hrubcová et al., 2017). On their way to the surface, fluids penetrate through faults, interact with the medium and are related to earthquake activity (Kämpf et al., 2013, 2019; Nickschick et al., 2015, 2019; Fischer et al., 2017; Liu et al., 2020). Subsurface life, protected from the intense radiation in the atmosphere, represents an ambience of the Earth's early biotopes; on the other hand, the microbial ecosystems abundant in the subsurface react to changes in the composition of fluids or to their long-term exposure.
Because of these phenomena (and their concentration in a rather small region), the West Bohemia–Vogtland (the western Eger Rift) area is a unique site worldwide and offers an ideal possibility for interdisciplinary study. The earthquake swarms and long-term degassing of mineral-rich waters and gases in granitic and sedimentary layers makes this area perfectly suited to study the fluid composition and fluid-induced source processes along with the effect of CO2 on the deep biosphere and the development of early life at depth. Long-term monitoring of the smallest signals and trend changes is essential to understand these phenomena and their interactions. This points to establishing a new level of multidisciplinary investigation. A modern, comprehensive, high-resolution observatory at depth with unique multiparameter observation can interconnect fields of primary research; advance the interactions among earthquakes, fluids, rocks and the biosphere; and contribute to answering related questions.
The seismicity in West Bohemia–Vogtland, also documented in macroseismic observations, occurs in the form of earthquake swarms, with the largest local magnitudes not exceeding ML 5 (e.g., 1875 or 1908). The largest instrumentally recorded earthquake occurred in the 1985–1986 swarm and reached a magnitude of ML 4.6 (Vavryčuk, 1993). Since 1985, seismicity has been concentrated in the Nový Kostel (NK) focal zone, where more than 80 % of seismic energy has been released within the last 30 years (Fischer and Michálek, 2008). The seismicity is generally shallow, with the event hypocenters occurring in the upper and middle crust, mainly between 5 and 15 km (Horálek and Fischer, 2008). Since 1997, the seismicity cluster beneath NK has been slowly but steadily growing, laterally more to the north and upwards, along a nearly planar structure (see Fig. 2).
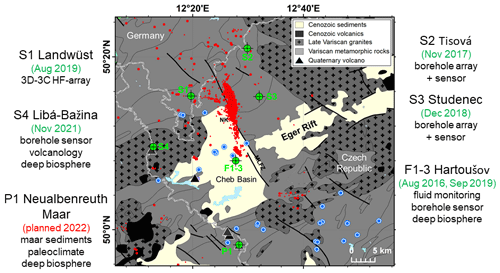
Figure 2The drilling sites and their prime scientific targets: S1 – Landwüst, S2 – Tisová, S3 – Studenec (seismological monitoring), S4 – Libá (seismological monitoring, volcanology and the deep biosphere in volcanic maar crater), F1–3 – Hartoušov (fluid, earthquake and deep-biosphere monitoring) and P1 (a planned borehole for paleoclimate and biosphere investigation). The time of realization is indicated in parentheses. Red circles represent the seismicity of the main Nový Kostel (NK) focal zone; blue circles represent mofettes and mineral springs with a gas discharge of more than 1 L min−1; Quaternary volcanoes are indicated by black triangles.
The surface earthquake recordings are of excellent quality (e.g., Fischer et al., 2014); however, they suffer from high-frequency wave damping by the near-surface weathered layers and site scattering, resulting in a smoothing of the wave signal details. A monitoring depth of a few hundred meters will avoid high-frequency attenuation and significantly improve the possibility of studying low-magnitude earthquakes in the crust and of resolving small-scale heterogeneities. This offers the possibility of analyzing the fluid-induced source processes and the anatomy of earthquake swarms and their migration in unprecedented detail. The fact that earthquake swarms in the Eger Rift region occur regularly and persistently in known spots of activity with known radiation patterns offers the opportunity to design and tune a borehole-based monitoring network for optimized analysis.
Systematic fluid probing and analyses at springs and mofettes in the western Eger Rift region have been performed for decades (Weise et al., 2001; Bräuer et al., 2003, 2005a, b, 2008, 2014; Schuessler et al., 2016). The results of the fluid isotope systematic monitoring have been capable of detecting trend changes in 3He 4He, which may indicate silent magmatic intrusions in the lower crust and upper mantle (e.g., Bräuer et al., 2009; Kämpf et al., 2013). However, the data obtained have not been sufficient to reach the objectives. The well-studied sites of massive CO2 degassing in mofettes offer the possibility of building a new generation of continuous real-time fluid monitoring systems at different depth levels. Such monitoring can separate the effects of surface and deep processes related to the composition and rate of fluids. It can also demonstrate the correlations between isotope composition and seismic activity (e.g., Bräuer et al., 2003, 2008, 2014) or reveal the link between an earthquake swarm and microbial activities (e.g., Bräuer et al., 2008).
Microbiological studies show the existence of diverse and active microbial ecosystems in the deep subsurface (e.g., Parkes et al., 1994, 2000; Lehman, 2007). This is a vast ambience, as between 75 % and 94 % of all microbes on the Earth occur in deeply buried marine and terrestrial sediments (Kallmeyer et al., 2012). Moreover, the deep subsurface harbors a huge carbon reservoir, equivalent to that of all plants on the Earth; thus, deeply buried microbial communities are very important for driving carbon and nutrient cycling as well as catalyzing a multitude of reactions among sediments, rocks and fluids. The Eger Rift area hosts a diverse lithology of surficial sediments overlying crystalline rocks as well as active CO2 degassing and high flow rates of mineral-rich fluids and gases (e.g., methane). The first studies of its mineral water and fluids (Alawi et al., 2015; Schuessler et al., 2016; Krauze et al., 2017; Liu et al., 2018, 2020) have indicated that the active fault systems of the Eger Rift area can be classified as a “hot spot” for microbial subsurface life. Microbial ecosystems abundant in the subsurface may react to changes in the composition of fluids. Thus, the long-term degassing of mineral-rich waters and gases in granitic and sedimentary layers makes this area ideally suited to study the effect of CO2 on the deep biosphere and the development of life at depth (Bussert et al., 2017). The maar-diatreme volcanos, as paleoconduit structures, are considered to be important pathways of magmatic fluids to study past activities under conditions in which the first biological molecules and later the first life forms originated (Schreiber et al., 2012). Thus, the Eger Rift area provides an environment for geo-microbiological studies and studies on the origin of deep life.
Such goals can be fulfilled by the development of a modern, comprehensive laboratory at depth to study the interconnected areas of primary research. Specifically, such a laboratory comprises the novel concept of 3-D seismic arrays with a set of shallow boreholes in order to reach a new level of high-frequency, near-source and multiparameter observation of earthquake swarms, real-time fluid monitoring at different depths and related phenomena. Such a network brings a new high detection capability, which improves the earthquake and fluid recordings. This offers the possibility to study extremely low-magnitude earthquakes and analyze the fluid-induced source processes. Repeated fluid probing at the surface can be complemented by a new generation of continuous real-time fluid monitoring in a safe and logistically accessible area. The variability in the local geological site conditions can meet the interdisciplinary targets for volcanologic, microbiological and paleoclimate research.
This initiative was introduced and discussed by Dahm et al. (2013) during the second ICDP Eger Rift workshop, resulting in a conceptual drilling approach to address the key scientific questions related to these processes. It was discussed among approximately 50 scientists from Germany, the Czech Republic, the USA, the UK and Poland; from these scientists, three scientific groups were identified based on their interests: (i) a seismological group, (ii) a fluid group, and (iii) a group interested in volcanology/petrology, paleoclimate and microbiology. Although each group is responsible for its field, together they comprise the unique interdisciplinary laboratory with a potential to better understand the following:
-
fluid–rock interactions and the mechanism of fluid-induced earthquake swarms,
-
the structure of fluid pathways from the upper mantle to the surface,
-
physical, chemical, and biological interrelations between geological processes, mantle-derived fluids and the biosphere down to 400 m depth,
-
the “fault-valve” mechanism and its relevance for earthquake triggering, seismic hazard, degassing and the activity of the deep biosphere,
-
the impact of CO2-rich mantle-derived fluids on the geo–bio interaction in the western Eger Rift,
-
the Quaternary paleoclimate and volcanic activity in the western Eger Rift region.
The in situ Eger comprehensive laboratory is currently being established by the International Continental Scientific Drilling Program (ICDP) in the framework of the interdisciplinary project “Drilling the Eger Rift: Magmatic Fluids Driving the Earthquake Swarms and the Deep Biosphere (EGER)”. Specifically, this laboratory at depth comprises a set of five new, distributed, shallow (less than 500 m deep) boreholes (Fig. 2). The drilling sites were selected to be distributed around the Nový Kostel (NK) focal zone; geophysical and geological surveys contributed to the selection of the exact locations. The drill holes are denoted S1–S4 (seismological monitoring) and F1–F3 (fluid monitoring), indicating the primary field of interest of each well (Fig. 2). The planned drill hole P1 will be the main record for paleoclimate studies.
The drillings S1–S4 are designed for seismological monitoring in order to reach a new level of high-frequency, near-source observations of earthquake swarms and related phenomena, like seismic noise and tremors generated by fluid movements. The drilling of S1 (Landwüst, depth 402 m), which is the only drill hole located in German territory, was completed in August 2019 and is supplemented by a 3-D high-frequency seismic array. The S2 site (Tisová, depth 460 m) was finished in November 2017, and the S3 site (Studenec, depth 408 m) was completed in December 2018; both S2 and S3 are planned with borehole seismic arrays. The drilling of S4 (Libá, depth 400 m) was accomplished in December 2021 in the recently discovered maar crater near the Czech–German border and will be equipped with a borehole seismometer.
The drill holes F1–F3 are primary designed for fluid monitoring in the framework of a multilevel gas monitoring system built in the Hartoušov mofette field. This mofette represents a gas emission site where CO2 ascends through crustal-scale conduits from as deep as the upper mantle; thus, the site can provide a natural window into ongoing magmatic processes at the mantle depth level. It is located at the crossing of the Eger Rift with the Počátky–Plesná zone (PPZ) tectonic lineament, which is the fault possibly related to the main Nový Kostel focal zone (Fig. 1). In particular, two existing monitoring wells, F1 and F2 (Bussert et al., 2017; Fischer et al., 2020), were complemented by the F3 drill hole; these three adjacent boreholes, F1 (30 m), F2 (70 m) and F3 (230 m), provide continuous monitoring of fluids at high sampling rates to acquire fluid parameters (gas flow, water temperature and water level/pressure) as well as chemical (CO2, Ar, N2, O2, He, H2 and CH4) and isotopic (δ13C, δ18O and 222Rn) gas content (Woith et al., 2020). Additionally, samples for laboratory analysis of He, Ne and Ar isotopes are taken repeatedly (roughly every 2 months), as theses isotopes are useful tracers for constraining the fluid origins and mixing ratios of mantle components. Moreover, the fluid monitoring at different depths separates the effects of surface and deep processes related to the composition and ascent rate of fluids. The drill site is also prepared for seismological monitoring to complement the monitoring network.
All boreholes were cored; the coring in Tertiary–Quaternary sedimentary sequences (S4, F2 and F3) is utilized for paleoclimate research and microbiological investigation (Bussert et al., 2017). The coring of solid rocks outcropping at the surface (S1 and S3, phyllites) is utilized for structural and tectonic investigation. Moreover, the core of drill hole S4, located in the maar crater, also targets volcanology and the evaluation of the neotectonic evolution of the maar. The final supplementary borehole, P1 (∼ 150 m), in another maar volcano near Neualbenreuth, Germany, at the Czech–German border is planned to support paleoclimate research and is scheduled for early 2023.
4.1 Pre-drilling site surveys
Several geophysical experiments were conducted to map deeper and shallow crustal structure. From reflection and seismic source data, distinct and highly reflective features (bright spots) were found in the upper crust close to the main NK focal zone (Mullick et al., 2015; Hrubcová et al., 2016). Local earthquake tomography showed clear indications of a mid-crustal intrusive body beneath the NK focal zone from increased P-wave S-wave (Vp Vs) ratios (Alexandrakis et al., 2014; Mousavi et al., 2015). Magnetotelluric investigations found highly conductive channel-like structures above the focal zone (Muñoz et al., 2018) that were complemented by highly attenuating bodies beneath and north of NK to 11 km depth (Mousavi et al., 2017). All of these features point to fluid pathways and interconnections between seismicity and fluid degassing.
The exact positions of the drill holes were investigated by local geophysical surveying to control the quality of waveforms, the signal-to-noise ratio (SNR), and to provide structural and geological constraints for fluid pathways and their movements. This comprised electric resistivity tomography and high-resolution reflection and refraction seismic surveying along the resistivity profile, as well as seismic noise measurements (Umlauft and Korn, 2019) at the Hartoušov site, both completed in late 2017 (Nickschick et al., 2019).
4.2 Drilling and coring
The drill sites are located in a natural mineral spring and spa resource protection zone and required specific permissions to meet the strict governmental requirements prior to the commencement of work. Drilling works were performed by the German drilling company Pruy KG with the HD110 drilling rig (the S1 site and the pre-drill of F3) and the Czech drilling company Geoněmec – vrty, s.r.o. with the Christensen 140C drilling rig (sites S2–S4). The drilling of F3 was conducted within the Swedish national research infrastructure for scientific drilling (Riksriggen) at Lund University, Sweden, with the Atlas Copco CT20C drilling rig (crawler mounted). Due to potential CO2 blowouts in the region, the drillings were performed through a blowout preventer to overcome the problem of pressurized CO2 in the drilling shaft; however, none of the sites faced such issues during drilling. The drillings were conducted under strict site contamination control conditions; after the termination of works, all sites and access roads were restored (Fig. 3). Furthermore, the wellheads were secured by a concrete head casing, and the cased peduncle was secured by a lock (Fig. 4).
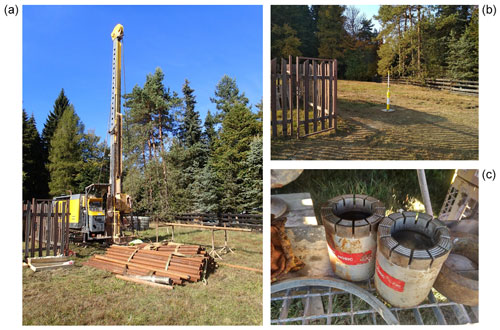
Figure 3The Eger Rift drilling setup, showing (a) the drilling rig with drilling rods at the S3 site, (b) the wellhead after the termination of work at the S3 site and (c) an example of a diamond drill head designed for drilling in hard rocks.
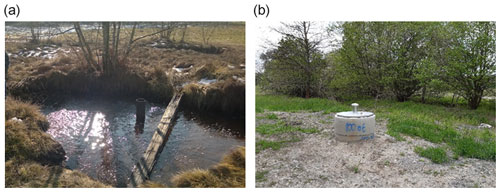
Figure 4Panel (a) shows the Hartoušov mofette field, and panel (b) presents the F3 borehole in Hartoušov after the completion of drilling works, with the concrete head casing secured by a lock.
The parameters of the drilling sites are summarized in Table 1. Some innovative approaches were applied during drilling and subsequent logging to meet the specific requirements of multidisciplinary research. All boreholes are nearly vertical, reaching depths < 460 m, and they were all steel cased. After casing, the drillings were cleared (redrilled within the casing) to ensure clear passage from the head to the bottom, which is necessary for subsequent successful installation of fluid and seismic monitoring instrumentation. Seismological drill holes S1, S3 and S4 were cemented to ensure seismic coupling. The quality of the cementing was controlled by well logging, and an innovative approach using a fiber-optic cable was additionally applied to monitor the cementing at S1. Wireline coring was applied to all boreholes, and the cores were retrieved and organized in wooden boxes (Fig. 5) before being stored (except for S2) at the Research INfrastructure for Geothermal ENergy (RINGEN) center in Litoměřice and the Federal Institute for Geosciences and Natural Resources (BGR) core repository in Spandau, Berlin (S1). The core of drill hole S2 is not available to the scientific community, as the drill hole was an in-kind contribution from the Golden Pet s.r.o. exploration company to the ICDP Eger project.
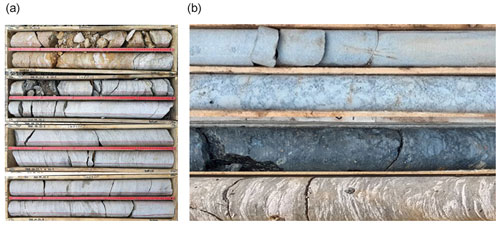
Figure 5Panel (a) presents an example of drill cores stored in wooden boxes that have been marked and labeled, showing phyllitic core from S1. Panel (b) presents an example of different lithologies in hard rocks, showing (from top to bottom) granite from S4, granite from S4, basalt from S4 and phyllite from S3.
Well logging was provided for all drilling sites except for S2 (the in-kind contribution from the Golden Pet s.r.o. exploration company). It comprised a complex of methods, including borehole geometry, caliper, sonic logging with full waveforms, and acoustic images to localize cracks, fractures, and/or tectonic and geological features. Gamma–gamma logging was applied to sample rock densities, neutron–neutron logging was applied for porosity and water content, natural gamma logging was used for the detection of unstable isotopes, and resistivity logging was used for the degree of rock deformation. For the records of well logging in individual boreholes, see Sect. 5.
Due to the specific requirements of microbiological research, drillings F3 and S4 were conducted under strict contamination control conditions, following the approach of Bussert et al. (2017). In the case of F3, the cores were retrieved in 3 m long polyvinyl chloride (PVC) liners to protect them from biological contamination; in the case of S4, the cores were encapsulated in aluminum. In both cases, the microbiological samples were frozen at −80 ∘C and sent for further analyses.
4.3 Seismological drillings – high-frequency 3-D arrays
Seismological monitoring requires the drillings to be distributed and optimized for detection, location, source mechanism and seismic wave scattering studies. The technical objectives of being able to analyze earthquakes (and nonvolcanic tremors) need to be addressed by taking a step up from the current short-period seismic monitoring network to a high-frequency 3-D seismic array. Boreholes S1–S3 involve deploying vertical seismic arrays combined with a surface small-aperture high-frequency array; a pilot observatory has already been deployed at S1. Such a configuration allows for detailed high-resolution study (at a 1 kHz sampling rate) of earthquake migration, short-term anomalies in the beginning phase of swarms, mixed-mode rupture processes, near-source scatterers, the depth distribution of events and the detection of microearthquakes along fluid channels. The location in unaltered rocks not affected by fluid ascent assures the recording of high-frequency signals of the smallest earthquakes () and is supported by the pioneering tests of Hiemer et al. (2012) from a small-aperture array of short-period stations at the surface (the 6-month eight-sensor test-array deployment near S1 at Rohrbach borehole V01–V08 detecting microearthquakes from from the NK focal zone).
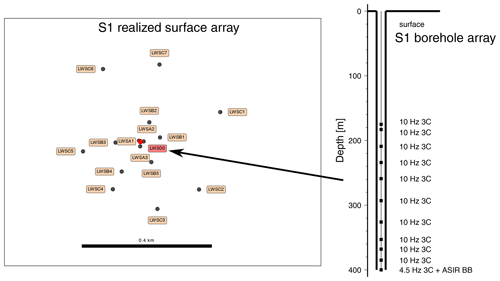
Figure 6Surface and borehole 3-D high-frequency seismological arrays and the types of sensors realized at the S1 site. Red color denotes the S1 borehole with a surface sensor.
Borehole S1 (depth 402 m, inner diameter (i.d.) 92 mm) is located in Landwüst (Germany) about 10 km northwest of the Nový Kostel (NK) focal zone in a forest area with basement rocks outcropping at the surface (metamorphosed Cambrian sediments – phyllites with quartzite layers). The test array installations and test measurements in the nearby Bad Brambach 80 m hole indicated the appropriate site conditions and excellent signal-to-noise ratios (SNRs) for weak microearthquakes with a significantly reduced S-wave damping effect. The S1 instrumentation comprises the ASIR bottom-hole broadband seismometer and a vertical array of 3C borehole sensors between 180 and 400 m; additionally, a surface array of 3C seismic sensors is installed around the borehole (Fig. 6). The fiber-optic cable was cemented behind the borehole casing to monitor the microearthquakes as well as the quality of cementing.
Borehole S2 (depth 464 m, i.d. 76 mm) is located near Tisová (Czech Republic) about 15 km north of the Nový Kostel (NK) focal zone in weakly metamorphosed Ordovician sediments (phyllites). The thickness of the overlying weathering products (debris with rock fragments) did not exceed several meters. The noise characterization from a test array deployed indicates good SNR conditions. The instrumentation, array design and configuration are planned to be the same as for borehole S1, except for the fiber-optic cable.
Borehole S3 (depth 400 m, i.d. 76 mm) is located in Studenec (Czech Republic) about 7 km northeast of the Nový Kostel (NK) focal zone in a dynamic landscape with a minimally weathered uppermost crust on metamorphosed Cambrian sediments (phyllites). The site is in a remote area with a good SNR; this is confirmed by long-term monitoring, as the site also coincides with one seismic station (STC) of the WEBNET surface monitoring network (Horálek et al., 2000). Moreover, this coincidence provides the opportunity to compare results from both networks and use the existing operation hut. A Güralp Radian bottom-hole seismometer (Güralp Co.) is currently installed and tested at the hole bottom.
4.4 Maar drilling – seismic monitoring, paleovolcanic and microbiological research
Borehole S4 (depth 406 m, i.d. 77 mm) is located in Bažina near Libá (Czech Republic), about 17.5 km southwest of the Nový Kostel (NK) focal zone, in a newly discovered volcanic maar structure penetrating the surrounding granitic rocks. The borehole sits in a conic maar crater and penetrates the alternating Quaternary siliciclastic and highly organic sediments of the crater. At 60 m depth, it reaches the basaltoids inside the crater; at a depth of 170 m, it reaches the contact of the crater with the surrounding granitoids (the host rocks), which continue until the bottom at 400 m depth. Because of its uppermost volcanic character, borehole S4 provides the record for combined paleovolcanic, magmatic, paleoclimate and deep-biosphere studies. As a detailed geophysical pre-site survey indicated good SNR conditions in granites, a bottom-hole seismometer is planned to be deployed in the solid granitic rocks at the bottom of the hole, along with a surface reference station.
4.5 Fluid and seismic monitoring and microbiological research – continuous sampling at different depths
Boreholes F1–F3 are located in the Hartoušov mofette field (Figs. 4, 7) in the Tertiary–Quaternary sedimentary successions of the Cheb Basin. The site appears well suited to exploring the relation between the swarm seismicity and CO2 degassing, as a massive coseismic increase in CO2 release has been observed here twice – in the case of the 2008 and 2014 earthquake swarms (Fischer et al., 2017). Three adjacent boreholes, F1 (depth 28 m, i.d. 115 mm), F2 (depth 108 m, i.d. 100 mm) and F3 (depth 239 m, i.d. 78 mm), supplemented by measurements in the nearby mofette (Fig. 7) allow continuous fluid monitoring at different depth levels within the basin sediments or in the CO2-permeated weathered crystalline basement (F3). The site survey comprised seismometers and a weather station installed on-site in order to quantify the impact of earthquakes as well as the environmental effects on the fluid regime. The multilevel gas monitoring system is being installed at three wells tapping the CO2 horizons at 20, 65 and 229 m. Continuous radon measurements while drilling revealed a promising CO2 horizon, which was later chosen for perforations of the steel casing. Further hydraulic tests at F3 are needed to confirm whether the perforation was successful.
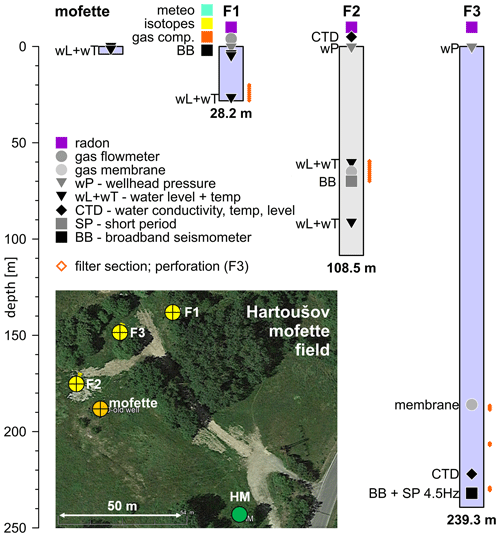
Figure 7Fluid and seismic monitoring in the Hartoušov mofette field (after Woith et al., 2020), showing boreholes F1–F3 and a mofette site with the sensors and types of monitoring. BB denotes a broadband seismometer (borehole and/or surface), and SP represents a short-period 4.5 Hz 3C borehole sensor.
The F2 borehole already hosts the ASIR broadband SiA seismometer at 70 m depth. Ultimately, a borehole seismometer will be installed at the bottom of F3 and will be complemented by a capillary tube to collect “fresh” gases from the CO2 horizon at depth, directly at the point where the fluids enter the borehole to avoid possible contamination or impact from external processes. Further details on the instrumentation of this mofette field with massive CO2 degassing (up to 97 t d−1) as well as the first monitoring results are summarized in Fischer et al. (2020), Woith et al. (2020) and Daskalopoulou et al. (2021). Once the novel monitoring system is fully operational, fluid transients will be able to be observed in great detail. We expect new insights into the physical processes that control the complex interplay between earthquakes, deep degassing and permeability variations along the path to the surface.
Seismic monitoring in F1–F3, located about 9 km south of the Nový Kostel (NK) focal zone (Fig. 2), complements the network of shallow boreholes. Borehole F2 (108.5 m depth) is equipped with a broadband borehole seismometer at 70 m depth; a similar sensor will be installed at the bottom of F3 (239 m depth). Moreover, a broadband surface seismic station is installed at F1 (Fig. 7).
The microbiological investigation at the Hartoušov mofette field was accomplished at the F2 and F3 drill holes. The pilot hole, F2 (HJB-1), was drilled in spring 2016, after extensive pre-drill surveys to optimize the well location (Bussert et al., 2017). The drilling through a thin caprock-like structure triggered a CO2 blowout, indicating a CO2 pathway. Pumping tests revealed a Na–Ca–HCO3–SO4-type groundwater with a total mineralization of 5870 mg L−1, which is typical for the mineral waters of Františkovy Lázně Spa that is located about 8 km to the west of the drill site (Bussert et al., 2017). The first microbiological investigations included activity tests for microbial methane production, DNA extractions and cultivation experiments as well as testing of the microbial DNA extracted from samples (Bussert et al., 2017). These steps were supplemented by the investigation of the F3 borehole which involved testing for microbiological life on samples from different depths. Further analyses of F3 microbial samples are ongoing.
4.6 Drilling for paleoclimate and microbiological research
Borehole P1 is planned in the Neualbenreuth Quaternary maar structure (in Germany, at a site located about 3 km southeast of the Mýtina maar) down to ∼ 150 m depth. It will penetrate a succession of lake sediments of at least 100 m depth with varying lithologies, surrounded by Paleozoic metamorphic rocks and underlain by the diatreme. Borehole P1 will be the main record for paleoclimate studies, as it will overlap an existing 100 m drill core obtained in 2015 (Rohrmüller et al., 2017), thereby enabling the development of continuous time series. Apart from paleoclimate, the site will be exploited for microbiological and deep-biosphere studies. Due to groundwater protection issues, the well has to be closed after drilling. The geophysical pre-site survey of maar structures indicates that shallow sedimentary successions of maar craters are not suitable for high-frequency seismic monitoring; thus, the deployment of a seismic sensor in this borehole is questionable.
The downhole logging measurements for the Eger Rift project have been performed in four of five boreholes (S1 Landwüst, S3 Studenec, S4 Libá and F3 Hartoušov). In this paper, we present the results of three seismological boreholes: S1 Landwüst, well logged by BLM Company in 2019 (Fig. 8); S3 Studenec, well logged by Aquatest in 2018 (Fig. 9); and S2 Tisová, which has not been well logged and only a stratigraphy profile is provided (Fig. 10). The following downhole logging measurements have been acquired: gamma ray, neutron–neutron, density, resistivity, temperature, P- and S-wave velocity, focus electrical resistivity (FEL), electrical conductivity, caliper, borehole deviation, and borehole azimuth direction. All logging measurements have been depth matched using the gamma ray as the reference logging present in all sondes. As the other two boreholes, S4 and F3, are the subject of further focused research, their profiles are presented in individual studies. Moreover, all records of stratigraphy and well logging in boreholes S1–S4 and F3 are depicted in Fischer et al. (2022) and in the upcoming Operational Report.
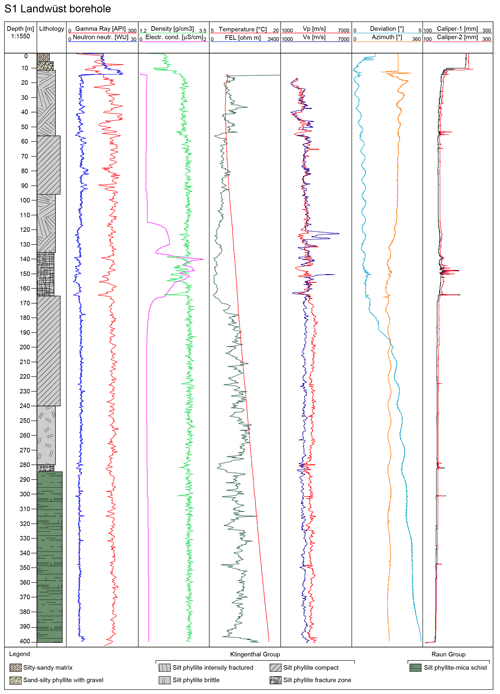
Figure 8Logging data of the S1 Landwüst borehole and a stratigraphy profile based on the initial core description. The abbreviations used in the figure are as follows: Neutron neutr. – neutron–neutron; WU – water unit; Electr. cond. – electrical conductivity; FEL – focus electrical resistivity; Vp and Vs – P- and S-wave velocity, respectively.
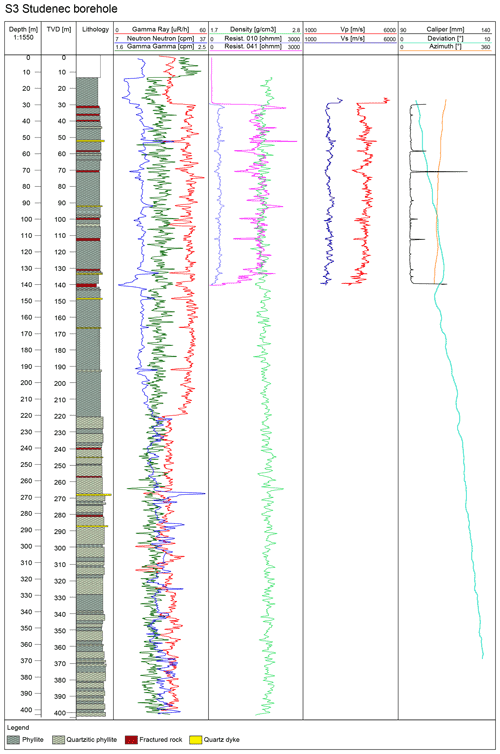
Figure 9Logging data of the S3 Studenec borehole and a stratigraphy profile based on the initial core description. The abbreviations used in the figure are as follows: TVD – total vertical depth; Resist. – resistivity; Vp and Vs – P- and S-wave velocity, respectively.
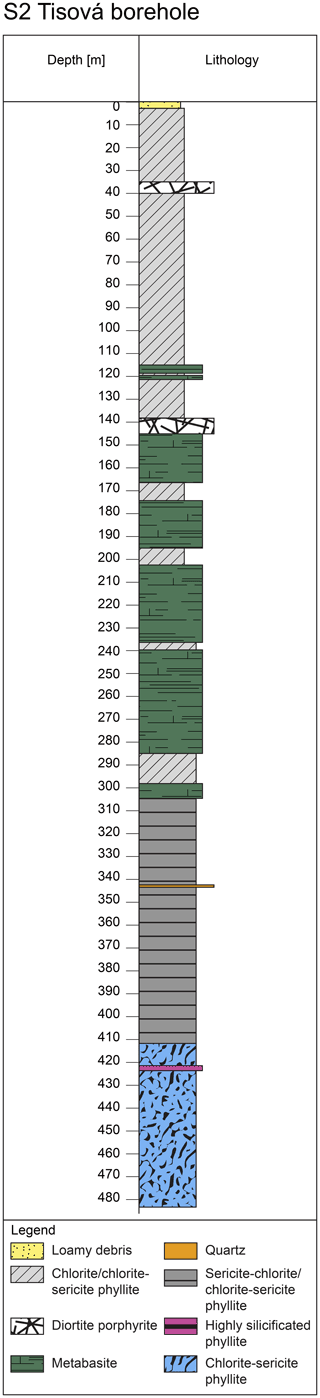
Figure 10Stratigraphy profile based on the initial core description of borehole S2 near Tisová in weakly metamorphosed Ordovician sediments (phyllites).
The S1 Landwüst borehole (Fig. 8) was drilled to 402 m depth and shows a southward deflection with a small deviation up to 5 ∘ at the bottom. The borehole encounters monotone silt–phyllite rocks with different stages of fracturing. All logs show a constant trend except at two main fractured intervals: one zone was identified from around 12.0 to 57.8 m, and the second zone was identified between 134 and 165 m. In these fractured zones, gamma ray, FEL, density, neutron–neutron, P- and S-wave velocity, and caliper logs show abrupt changes. In particular, in the second fracture interval, the electrical conductivity shows constant values of around 0.2 µS cm−1 up to 115 m that slightly increase from 0.2 to 1.8 µS cm−1 between 115 and 140 m. The fracturing becomes more intense between 140 and 155 m, where the electrical conductivity reaches the maximum values of around 1.8 µS cm−1; the values then gradually decrease to 0.7 µS cm−1 at 165 m before reaching a constant value of 0.2 µS cm−1 at the bottom. These two main fracture zones were also detected by the caliper, which indicates an opening from 155 to 200 mm. The temperature in the borehole increases continuously from 7 ∘C at 25 m to 13.5 ∘C at 400 m depth.
The S3 Studenec borehole (Fig. 9) reached a total depth of 402.5 m, with the deviation increasing smoothly up to 9 ∘ at the bottom and with deflection to the southeast. The borehole encounters a sequence of phyllite rocks up to 221 m, and a quartzitic phyllite formation is then predominant in the bottom part. The variation in lithology along the borehole is well recorded by the gamma ray log. The gamma ray shows average values of 50 µR h−1 for the phyllite rock formation, mainly related to the higher content of clay minerals compared with the quartzitic phyllite formation which shows average values around 39 µR h−1. A slight enrichment of the clay component is observed between 330 and 402.5 m. Around 139–141 m, the borehole encountered a tectonic fault that fragmented the rock of the borehole wall and caused the collapse of the section between 140 and 142.5 m. Only downhole logging measurements with nuclear sources (such as neutron–neutron and density measurements) have been run inside the pipe from 0 m to the bottom of the hole, whereas P- and S-wave velocity, caliper and resistivity logs were provided only in shallow part between 30 and 140 m. Several fracture intervals have been identified around 59, 71.0, 100, 111 and 135 m. They are detected by resistivity, P-wave velocity and gamma ray logs, showing a decrease in values compared with the general trend, whereas the caliper records show an increase in the hole size (spikes).
Due to the persistent tectonic activity and the existence of thermal springs, places with increased heat flux (Čermák, 1994) can be expected in the area. However, due to the absence of deeper boreholes penetrating sedimentary successions of the Cheb Basin to the basement crystalline rocks, detailed information is missing. For this reason, precise temperature well logging was performed in all boreholes (Fig. 11). The temperature recordings allowed for the determination of the temperature gradients, and topographic corrections were subsequently calculated for each well using 3-D numerical models. To determine the heat flow density, knowledge of the thermal conductivity of the rocks intersected by the boreholes was necessary. The thermal properties (thermal conductivity, thermal diffusivity and volumetric heat capacity) were measured using a high-resolution optical scanning method on several samples from each borehole. The anisotropy of the thermal properties was also assessed, which turned out to be very significant especially in the case of boreholes (S1, S2 and S3) intersecting the metamorphic rocks. Based on the above, we calculated the heat flow, which ranged from 75 mW m−2 for borehole S2 near Tisová to more than 100 mW m−2 for S1 in Landwüst.
The 3-D high-frequency seismic array at the S1 site has been accomplished as a pilot array for the seismic part of the ICDP Eger Rift project. The S1 instrumentation comprises the ASIR bottom-hole broadband seismometer and a vertical array of 3C borehole sensors between 180 and 400 m; additionally, a surface array of 3C seismic sensors is installed (Fig. 6). A fiber-optic cable was cemented outside of the borehole casing to monitor the microearthquakes and will provide comparison with the borehole chain. Moreover, it was also used to check the quality of the casing cementation.
7.1 Borehole array
The downhole array consists of two major parts: a 10-level high-frequency borehole chain and one additional bottom-hole seismometer. The borehole chain is assembled with high-frequency geophones for one vertical and two horizontal directions at 10 depth levels. We identified 10 Hz HG-7 geophones as the optimum sensors (little or no tilt sensitivity, high spurious and low corner frequencies and, thus, a large usable bandwidth). In order to increase the output voltage, the geophones are in sets of two sensors connected in series for each direction and depth level, which results in a total of 30 analogue channels to be handled. The analogue signals are converted by five six-channel Earth Data EDR-209 digitizers at a rate of (up to) 1 kHz. The bottom-hole equipment consists of a newly developed ASIR SiA broadband seismometer with a passband of 200 s to 1.5 kHz bundled with a 3C 4.5 Hz geophone. The analogue signals are converted by one six-channel Earth Data EDR-209 digitizer at a rate of (up to) 1 kHz. The example of waveforms of an ML 0.1 microearthquake recorded with a borehole chain at S1 Landwüst is presented in Fig. 12.
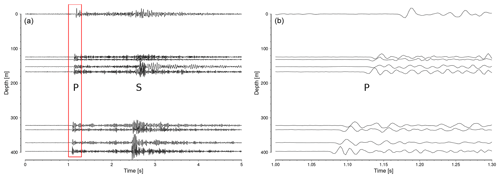
Figure 12Example of microearthquake waveforms recorded with an 8-level borehole chain at S1 Landwüst (test installation before the deployment of the final 10-level chain) for the event on 23 January 2020, 22:21:11 UTC at 8 km depth, ML 0.1, at an epicentral distance of 11 km (near Luby). Note the good signal-to-noise ratios (SNRs) of the recordings and their improvement with depth. The red box in panel (a) indicates the zoomed area in panel (b).
7.2 Surface array
A small-aperture array of short-period stations at the surface consists of three rings around a central borehole station. The aperture of the first ring is 10 m, and it comprises three 3 m deep postholes that are not yet fully equipped. The second ring has an aperture of 100 m and comprises five sensors. Ring 3 is equipped with seven sensors with an aperture of 400 m. The surface sensors are positioned in shallow (50 cm deep) holes and powered by solar panels. The sensors of rings 2 and 3 are of the same type (4.5 Hz HG-6 geophones with a gain of 27.7 Vs m−1). The sampling rate of 1 kHz is used with seismological data loggers (EDR-209) and near-real-time transmission of the data. The data are transmitted via mobile connection.
7.3 Fiber-optic cable – the quality of cementing
After drilling S1, a fiber-optic cable was installed behind the casing down to a depth of 397.5 m on the western side of the well. The fiber-optic cable had a tight buffer design. It contained one single-mode optical fiber (ITU-T G.652.D/657.A1) preserved in a stainless-steel metal tube and embedded in a polyamide sheath with a structured surface (outer diameter 3.2 mm) to increase the mechanical coupling between cement and cable. The cable was attached to the casing using cable ties and tape. Two fixations were used per tube joint. To improve the cement deposition and reduce the risk of micro-channels between cable and cement, 4 mm separators were installed between the cable and the casing. Figure 13a shows the fiber-optic cable (and its fixing with separators) prepared for the deployment in the well.
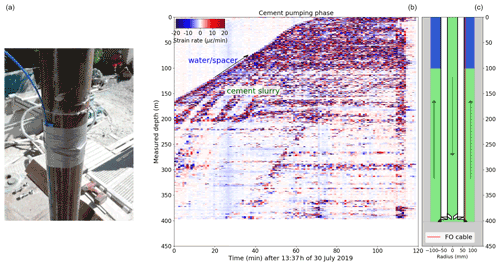
Figure 13Panel (a) shows the fiber-optic cable (blue) fixed at the 4.5 in. (115 mm) borehole casing of S1. Blue separators can be seen beneath the tape to improve the cable coupling. Panel (b) presents the fiber-optic distributed strain rate measurements during cementing of the 4.5 in. (115 mm) casing. Panel (c) provides a sketch of cementing (flow indicated by arrows); the cement suspension (green) and spacer fluid (blue) are schematically depicted for the time of 26 min.
To verify cable integrity during installation, optical time-domain reflectometry was performed after cable installation and prior to cementation. Afterwards, the well cementation was performed to ensure the best possible coupling of the casing and the surrounding rocks. The quality of cementation was controlled by the distributed fiber-optic strain measurements along the cable with an optical backscatter reflectometer (Luna OBR 4400) previously used in borehole applications (e.g., Lipus et al., 2018). The OBR interrogator is based on the optical frequency domain reflectometry (OFDR) principle, following Froggatt and Moore (1998). The measurement resulted in a strain section recorded every 60 s over a time span of 2 h during the cement pumping. An example of such measurement is depicted in Fig. 13b, showing the strain change between two successive measurements.
The ICDP Eger project comprises a large number of continuously operating sensors for recording seismic, gas-related and meteorological data streams. In future, additional sensors also are planned for the detection of infrasonic and/or rotational seismic signals. As the project represents a long-term monitoring effort and shall be operated for at least 10–15 years, a vast amount of data has to be considered.
The major part of these data is represented by seismic monitoring at the S1–S4 and F1–F3 sites, where the 3-D arrays at the S1–S3 sites play a particularly crucial role. Each of these sites, equipped with 25 geophones/seismometers (3C instruments in all cases) with both borehole (9 geophones and 1 seismometer) and surface installations (15 geophones), produces a data stream of 75 seismic channels. With the continuous sampling rate of 1 kHz, the amount of data produced at each 3-D 3C array installation at S1–S3 will be in the order of 16 GB d−1 (75 channels × 1000 samples (channel × s) × 86 400 s d−1 × 2.5 B per sample = 16.2 GB d−1). This sums to 50 GB d−1 (including S4), i.e., ∼ 1.5 TB every month accumulating to 180 TB over a 10-year operating period. Data streams will be transmitted to Potsdam and/or partner institutions in Prague and Leipzig via 4G mobile phone standard to an open VPN server with SeedLink. The amount of data produced from gas sensors and meteorological data will be much smaller and can safely be estimated to be in the order of 1 % of the seismic data.
The new in situ geodynamic laboratory established in the framework of the ICDP Eger project involves five borehole sites aimed at long-term monitoring of seismic activity and CO2 degassing in the West Bohemia–Vogtland geodynamic region (western Eger Rift). At each of these sites, a borehole broadband seismometer will be installed, and sites S1, S2 and S3 will also host a 3-D seismic array composed of a vertical geophone chain and surface seismic array. To date, all of the monitoring boreholes have been drilled. This includes the seismic monitoring boreholes S1, S2 and S3 in the crystalline units north and east of the major seismogenic zone of Nový Kostel (NK), borehole F3 in the Hartoušov mofette field south of NK and borehole S4 in the newly discovered Bažina maar near Libá west of NK. An additional borehole, P1, is being prepared in the Neualbenreuth maar, Germany, and is aimed at paleoclimate research. Seismic instrumenting has been completed in the S1 borehole and is under preparation at the four remaining monitoring borehole sites (S2, S3, S4 and F3). The continuous fluid monitoring site Hartoušov includes three boreholes, F1, F2 and F3, and a pilot monitoring phase is underway.
It is expected that the Eger Rift laboratory will result in an increasing sensitivity and discrimination capability with respect to seismic monitoring. Thanks to fluid sampling at different depths, which removes near-surface contamination, it will allow for enhanced monitoring of ongoing deep magmatic processes. This will improve our understanding of the relationships among mofette degassing, gas composition and swarms. The Eger Rift borehole laboratory also enables one to analyze microbial activity at CO2 mofettes and maar structures in the context of changes in habitats. Last but not least, drillings into the maar volcanoes contribute to a better understanding of the Quaternary paleoclimate and volcanic activity.
After a 3-year embargo period, beginning on the date of sampling party, the downhole logging and stratigraphy data will be available at the ICDP repository database (https://nextcloud.gfz-potsdam.de, last access: 7 June 2022; Fischer et al., 2022).
TF, TD, PH and HW designed, planned and executed the drillings and raised funding. TV executed the drillings and the coring. JH and JeK designed the drillings. MO, JV, PD, HK, MZ, MPL, FK, KH, TR, JaK, DV and KD performed the measurements. PH, TF, TD and HW wrote the manuscript, SP wrote the well-logging section, and all authors reviewed and edited the manuscript.
The contact author has declared that neither they nor their co-authors have any competing interests.
Publisher’s note: Copernicus Publications remains neutral with regard to jurisdictional claims in published maps and institutional affiliations.
The ICDP Eger Rift project was funded by the International Continental Scientific Drilling Program (ICDP); the Deutsche Forschungsgemeinschaft (DFG, German Research Foundation) – project nos. 419880416, 419459207 and 419909358; the Czech Science Foundation; GFZ Potsdam; a large project of the Czech infrastructure CzechGeo (CZ.02.1.01/0.0/0.0/16_013/0001800); and CzechGeo/EPOS (LM2015079). We also received co-funding from the Swedish National Research infrastructure for scientific drilling (Riksriggen) at Lund University, Sweden. The in-kind contribution of S2 drilling by the Golden Pet s.r.o. exploration company is gratefully acknowledged.
For technical support before and during drilling, we are grateful to Ulrich Harms, Thomas Wiersberg, Santiago Aldaz and Jochem Kück of the ICDP Operational Support Group. We thank the international team of drillers from Geoněmec – vrty, s.r.o., Protek Norr AB and PRUY KG, with special thanks to František Kalenda and Milan Němec (Czech Republic), Danilo Pruy (Germany), Johan Kullenberg (Sweden) and Friðfinnur K. Danielsson (Iceland). We thank also Ralf Bauz (Germany) for support during the drilling of S1 and F3.
We acknowledge Christian Cunow and Marius Isken for support during the S1 field and cable installations, and we are grateful to Marius Kriegerowski and Henning Lilienkamp for seismic noise measurements. Funding for the sensors and equipment for the S1 surface array was provided by the Saxionan State Office for Agriculture, Environment and Geology and the University of Leipzig. Special thanks go to LIAG (Germany) for well logging of F3 and Aquatest/SG Geotechnika (Czech Republic) for well logging of the other sites.
We also thank the editor Tomoaki Morishita, Ulrich Harms (the editor in chief) and the two anonymous reviewers for their comments that improved the paper.
This research has been supported by the International Continental Scientific Drilling Program (ICDP, project no. 5008); the Deutsche Forschungsgemeinschaft (DFG, German Research Foundation, project nos. 419880416, 419459207 and 419909358); the Czech Science Foundation; GFZ Potsdam; a large project of the Czech infrastructure CzechGeo (grant no. CZ.02.1.01/0.0/0.0/16_013/0001800); and CzechGeo/EPOS (no. LM2015079). We also received co-funding from the Swedish National Research infrastructure for scientific drilling (Riksriggen) at Lund University, Sweden, and an in-kind contribution of S2 drilling from the Golden Pet s.r.o. exploration company.
This paper was edited by Tomoaki Morishita and reviewed by two anonymous referees.
Alawi, M., Nickschick, T., and Kämpf, H.: Mikrobiologische Prozesse in CO2-Aufstiegskanälen, System Erde, 5, 28–33, https://doi.org/10.2312/GFZ.syserde.05.01.5, 2015.
Alexandrakis, C., Calò, M., Bouchaala, F., and Vavryčuk, V.: Velocity structure and the role of fluids in the West Bohemia Seismic Zone, Solid Earth, 5, 863–872, https://doi.org/10.5194/se-5-863-2014, 2014.
Bräuer, K., Kämpf, H., Strauch, G., and Weise, S. M.: Isotopic evidence (3He 4He, 13CCO2) of fluid triggered intraplate seismicity, J. Geophys. Res., 108, 2070, https://doi.org/10.1029/2002JB002077, 2003.
Bräuer, K., Kämpf, H., Faber, E., Koch, U., Nitzsche, H.-M., and Strauch, G.: Seismically triggered microbial methane production relating to the Vogtland NW Bohemia earthquake swarm period 2000, Central Europe, Geochem. J., 39, 441–450, https://doi.org/10.2343/geochemj.39.441, 2005a.
Bräuer, K., Kämpf, H., Niedermann, S. and Strauch, G.: Evidence for ascending upper mantle-derived melt beneath the Cheb basin, central Europe, Geophys. Res. Lett., 32, L08303, https://doi.org/10.1029/2004GL022205, 2005b.
Bräuer, K., Kämpf, H., Niedermann, S., Strauch, G., and Tesař, J.: The natural laboratory NW Bohemia – Comprehensive fluid studies between 1992 and 2005 used to trace geodynamic processes, Geochem. Geophy. Geosy., 9, Q04018, https://doi.org/10.1029/2007GC001921, 2008.
Bräuer, K., Kämpf, H., and Strauch, G.: Earthquake swarms in non-volcanic regions: What fluids have to say, Geophys. Res. Lett., 36, L17309, https://doi.org/10.1029/2009GL039615, 2009.
Bräuer, K., Kämpf, H., and Strauch, G.: Seismically triggered anomalies in the isotope signatures of mantle-derived gases detected at degassing sites along two neighbouring faults in NW Bohemia, Central Europe, J. Geophys. Res.-Sol. Ea., 119, 5613–5632, https://doi.org/10.1002/2014JB011044, 2014.
Bussert, R., Kämpf, H., Flechsig, C., Hesse, K., Nickschick, T., Liu, Q., Umlauft, J., Vylita, T., Wagner, D., Wonik, T., Flores, H. E., and Alawi, M.: Drilling into an active mofette: pilot-hole study of the impact of CO2-rich mantle-derived fluids on the geo–bio interaction in the western Eger Rift (Czech Republic), Sci. Dril., 23, 13–27, https://doi.org/10.5194/sd-23-13-2017, 2017.
Čermák, V.: Results of heat flow studies in Czechoslovakia, in Crustal structure of the Bohemian Massif and the West Carpathians, in: Exploration of the Deep Continental Crust, edited by: Bucha, V. and Blížkovský, M., Berlin, Springer-Verlag, 85–120, ISBN 3540579869, 1994.
Dahm, T. and Brandsdottir, B.: Moment tensors of micro-earthquakes from the Eyjafjallajökull volcano in South Iceland, Geophys. J. Int., 130, 183–192, 1997.
Dahm, T., Hrubcová, P., Fischer, T., Horálek, J., Korn, M., Buske, S., and Wagner, D.: Eger Rift ICDP: an observatory for study of non-volcanic, mid-crustal earthquake swarms and accompanying phenomena, Sci. Dril., 16, 93–99, https://doi.org/10.5194/sd-16-93-2013, 2013.
Daskalopoulou, K., Woith, H., Zimmer, M., Niedermann, S., Barth, J. A. C., Frank, A. H., Vieth-Hillebrand, A., Vlček, J., Bağ, C. D., and Bauz, R.: Insight into Hartoušov Mofette, Czech Republic: Tales by the Fluids, Front. Earth Sci., 9, 615766, https://doi.org/10.3389/feart.2021.615766, 2021.
Dreger, D. S., Tkalcic, H., and Jonston, M.: Dilational processes accompanying earthquakes in the Long Valley Caldera, Science, 288, 122–125, 2000.
Einarsson, P.: Earthquakes and present-day tectonism in Iceland, Tectonophysics, 189, 261–279, 1991.
Fischer, T. and Michálek, J.: Post 2000-swarm microearthquake activity in the principal focal zone of West Bohemia/Vogtland: space-time distribution and waveform similarity analysis, Stud. Geophys. Geod., 52, 493–511, https://doi.org/10.1007/s11200-008-0034-y, 2008.
Fischer, T., Horálek, J., Hrubcová, P., Vavryčuk, V., Bräuer, K., and Kämpf, H.: Intra-continental earthquake swarms in West-Bohemia and Vogtland: A review, Tectonophysics, 611, 1–27, https://doi.org/10.1016/j.tecto.2013.11.001, 2014.
Fischer, T., Matyska, C., and Heinicke, J.: Earthquake-enhanced permeability – evidence from carbon dioxide release following the ML 3.5 earthquake in West Bohemia, Earth Planet. Sci. Lett., 460, 60–67, https://doi.org/10.1016/j.epsl.2016.12.001, 2017.
Fischer, T., Vlček, J., and Lanzendörfer, M.: Monitoring crustal CO2 flow: methods and their applications to the mofettes in West Bohemia, Solid Earth, 11, 983–998, https://doi.org/10.5194/se-11-983-2020, 2020.
Fischer, T., Hrubcová, P., Dahm, T., Woith, H., Vylita, T., Ohrnberger, M., Vlček, J., Horálek, J., Dedeček, P., Zimmer, M., Lipus, M. P., Pierdominici, S., Kallmeyer, J., Krüger, F., Hannemann, K., Korn, M., Kämpf, H., Reinsch, T., Klicpera, J., Vollmer, D., and Daskalopoulou, K.: ICDP Drilling of the Eger Rift Observatory: Operational Data Sets, GFZ Data Services [data set], https://doi.org/10.5880/ICDP.5008.001, 2022.
Flechsig, C., Heinicke, J., Mrlina, J., Kämpf, H., Nickschick, T., Schmidt, A., Bayer, T., Günther, T., Rücker, C., Seidel, E., and Seidl, M.: Integrated geophysical and geological methods to investigate the inner and outer structures of the Quaternary Mýtina maar (W-Bohemia, Czech Republic), Int. J. Earth Sci., 104, 2087–2105, https://doi.org/10.1007/s00531-014-1136-0, 2015.
Froggatt, M. and Moore, J.: High-spatial-resolution distributed strain measurement in optical fiber with Rayleigh scatter, Appl. Optics, 37, 1735–1740, https://doi.org/10.1364/AO.37.001735, 1998.
Geissler, W. H., Kämpf, H., Kind, R., Klinge, K., Plenefisch, T., Horálek, J., Zedník, J., and Nehybka, V.: Seismic structure and location of a CO2 source in the upper mantle of the western Eger (Ohře) Rift, central Europe, Tectonics, 24, TC5001, https://doi.org/10.1029/2004TC001672, 2005.
Grünthal, G., Stromeyer, D., Bosse, C., Cotton, F., and Bindi, D.: Erdbebengefährdung Deutschlands – neu bewertet für aktuelle Baunorm, System Erde, 9, 1, 26–31, https://doi.org/10.2312/GFZ.syserde.09.01.4, 2019.
Hiemer, S., Roessler, D., and Scherbaum, F.: Monitoring the West Bohemian earthquake swarm in 2008/2009 by a temporal small-aperture seismic array, J. Seismol., 16, 169–182, https://doi.org/10.1007/s10950-011-9256-5, 2012.
Horálek, J. and Fischer, T.: Role of crustal fluids in triggering the West Bohemia/Vogtland earthquake swarms: just what we know (a review), Stud. Geophys. Geod., 52, 455–478, https://doi.org/10.1007/s11200-008-0032-0, 2008.
Horálek, J., Fischer, T., Boušková, A., and Jedlička, P.: The Western Bohemia/Vogtland Region in the light of the Webnet network, Stud. Geophys. Geod., 44, 107–125, https://doi.org/10.1023/A:1022198406514, 2000.
Hrubcová, P. and Geissler, W. H.: The crust-mantle transition and the Moho beneath the Vogtland/West Bohemian region in the light of different seismic methods, Stud. Geophys. Geod., 53, 275–294, https://doi.org/10.1007/s11200-009-0018-6, 2009.
Hrubcová, P., Środa, P., Špičák, A., Guterch, A., Grad, M., Keller, G.R., Brückl, E., and Thybo, H.: Crustal and uppermost mantle structure of the Bohemian Massif based on CELEBRATION 2000 data, J. Geophys. Res., 110, B11305, https://doi.org/10.1029/2004JB003080, 2005.
Hrubcová, P., Vavryčuk, V., Boušková, A., and Horálek, J.: Moho depth determination from waveforms of microearthquakes in the West Bohemia/Vogtland swarm area, J. Geophys. Res.-Sol. Ea., 118, 120–137, https://doi.org/10.1029/2012JB009360, 2013.
Hrubcová, P., Vavryčuk, V., Boušková, A., and Bohnhoff, M.: Shallow crustal discontinuities inferred from waveforms of microearthquakes: Method and application to KTB Drill Site and West Bohemia Swarm Area, J. Geophys. Res.-Sol. Ea., 121, 881–902, https://doi.org/10.1002/2015JB012548, 2016.
Hrubcová, P., Geissler, W. H., Bräuer, K., Vavryčuk, V., Tomek, Č., and Kämpf, H.: Active magmatic underplating in western Eger Rift, Central Europe, Tectonics, 36, 2846–2862, https://doi.org/10.1002/2017TC004710, 2017.
Kallmeyer, J., Pockalny, R., Adhikari, R. R., Smith, D. C., and D'Hondt, S.: Global distribution of microbial abundance and biomass in subseafloor sediment, P. Natl. Acad. Sci. USA, 109, 16213–16216, 2012.
Kämpf, H., Bräuer, K., Schumann, J., Hahne, K., and Strauch, G.: CO2 discharge in an active, non-volcanic continental rift area (Czech Republic): characterisation (δ13C, 3He 4He) and quantification of diffuse and vent CO2 emissions, Chem. Geol., 339, 71–83, https://doi.org/10.1016/j.chemgeo.2012.08.005, 2013.
Kämpf, H., Broge, A. S., Marzban, P., Allahbakhshi, M., and Nickschick, T.: Nonvolcanic carbon dioxide emission at continental rifts: the Bublak Mofette Area, Western Eger Rift, Czech Republic, Geofuids, 2019, 1–19, https://doi.org/10.1155/2019/4852706, 2019.
Klemt, C.: Seismic imaging of the crustal structure in the central European Variscan orogen by reprocessing of the deep seismic reflection profiles GRANU9501 und GRANU9502, MS thesis, TU Bergakademie, Freiberg, 2013.
Krauze, P., Kämpf, H., Horn, F., Liu, Q., Voropaev, A., Wagner, D., and Alawi, M.: Microbiological and geochemical survey of CO2-dominated mofette and mineral waters of the Cheb Basin, Czech Republic, Front. Microbiol., 8, 2446, https://doi.org/10.3389/fmicb.2017.02446, 2017.
Kvaček, Z. and Teodoridis, V.: Tertiary macrofloras of the Bohemian Massif: a review with correlations within Boreal and Central Europe, Bull. Geosci., 82, 383–408, 2007.
Lees, J. M.: Multiplet analysis at Coso geothermal, Bull. Seismol. Soc. Am., 88, 1127–1143, 1998.
Lehman, R. M.: Microbial distribution and their potential controlling factors in terrestrial subsurface environments, in: The spatial distribution of microbes in the environment, edited by: Franklin, R. B. and Mills, A. L., Springer, 135–178, https://doi.org/10.1007/978-1-4020-6216-2, 2007.
Lied, P., Kontny, A., Nowaczyk, N., Mrlina, J., and Kämpf, H.: Cooling rates of pyroclastic deposits inferred from mineral magnetic investigations: a case study from the Pleistocene Mýtina Maar (Czech Republic), Int. J. Earth Sci., 109, 1707–1725, https://doi.org/10.1007/s00531-020-01865-1, 2020.
Lipus, M. P., Reinsch, T., Schmidt-Hattenberger, C., Henninges, J., and Reich, M.: Gravel pack monitoring with a strain sensing fiber optic cable, Oil Gas-Eur. Mag., 44, 179–185, https://doi.org/10.19225/181202, 2018.
Liu, Q., Kämpf, H., Bussert, R., Krauze, P., Horn, F., Nickschick, T., Plessen, B., Wagner, D., and Alawi, M.: Influence of CO2 degassing on the microbial community in a dry mofette field in Hartoušov, Czech Republic (Western Eger Rift), Front. Microbiol., 9, 2787, https://doi.org/10.3389/fmicb.2018.02787, 2018.
Liu, Q., Adler, K., Lipu, D., Kämpf, H., Bussert, R., Plessen, B., Schulz, H.-M., Krauze, P., Horn, F., Wagner, D., Mangelsdorf, K., and Alawi, M.: Microbial Signatures in Deep CO2-Saturated Miocene Sediments of the Active Hartoušov Mofette System (NW Czech Republic), Front. Microbiol., 11, 543260, https://doi.org/10.3389/fmicb.2020.543260, 2020.
Mousavi, S., Bauer, K., Korn, M., and Hejrani, B.: Seismic tomography reveals a mid-crustal intrusive body, fluid pathways and their relation to the earthquake swarms in West Bohemia/Vogtland, Geophys. J. Int., 203, 1113–1127, https://doi.org/10.1093/gji/ggv338, 2015.
Mousavi, S., Haberland, C., Bauer, K., Hejrani, B., and Korn, M.: Attenuation tomography in West Bohemia/Vogtland, Tectonophysics, 695, 64–75, https://doi.org/10.1016/j.tecto.2016.12.010, 2017.
Mrlina, J., Kämpf, H., Geissler, W. H., and van den Bogaard, P.: Proposed Quaternary maar structure at the Czech/German boundary between Mýtina and Neualbentreuth (western Eger Rift, Central Europe): geophysical, petrochemical and geochronological indications, Z. Geol. Wiss., 35, 213–230, 2007.
Mrlina, J., Kämpf, H., Kroner, C., Mingram, J., Stebich, M., Brauer, A., Geissler, W. H., Kallmeyer, J., Matthes, H., and Seidl, M.: Discovery of the first Quaternary maar in the Bohemian Massif, Central Europe, based on combined geophysical and geological surveys, J. Volcanol. Geoth. Res., 182, 97–112, https://doi.org/10.1016/j.jvolgeores.2009.01.027, 2009.
Mullick, N., Buske, S., Hrubcová, P., Růžek, B. Shapiro, S., Wigger, P., and Fischer, T.: Seismic imaging of the geodynamic activity at the western Eger rift in central Europe, Tectonophysics, 647–648, 105–111, https://doi.org/10.1016/j.tecto.2015.02.010, 2015.
Muñoz, G., Weckmann, U., Pek, J., Kováčiková, S., and Klanica, R.: Regional two-dimensional megnetotelluric profile in West Bohemia/Vogtland reveals deep conductive channel into the earthquake swarm region, Tectonophysics, 727, 1–11, https://doi.org/10.1016/j.tecto.2018.01.012, 2018.
Nickschick, T., Kämpf, H., Flechsig, C., Mrlina, J., and Heinicke, J.: CO2 degassing in the Hartousov mofette area, western Eger Rift, imaged by CO2 mapping and geoelectrical and gravity surveys, Int. J. Earth Sci., 104, 2107–2129, https://doi.org/10.1007/s00531-014-1140-4, 2015.
Nickschick, T., Flechsig, C., Mrlina, J., Oppermann, F., Löbig, F., and Günther, T.: Large-scale electrical resistivity tomography in the Cheb Basin (Eger Rift) at an International Continental Drilling Program (ICDP) monitoring site to image fluid-related structures, Solid Earth, 10, 1951–1969, https://doi.org/10.5194/se-10-1951-2019, 2019.
Parkes, R. J., Cragg, B. A., Bale, S. J., Getliff, J. M., Goodman, K., Rochelle, P. A., Fry, J. C., Weightman, A. J., and Harvey, S. M.: Deep bacterial biosphere in Pacific Ocean sediments, Nature, 371, 410–413, 1994.
Parkes, R. J., Cragg, B. A., and Wellsbury, P.: Recent studies on bacterial populations and processes in subseafloor sediments: a review, Hydrogeol. J., 8, 11–28, 2000.
Prodehl, C., Mueller, S., and Haak, V.: The European Cenozoic Rift System, in: Continental rifts: evolution, structure, tectonics, in: Dev. Geotecton, edited by: Olsen, K. H., Elsevier, 133–212, ISBN 9780080529837, 1995.
Rohrmüller, J., Kämpf, H., Geiß, E., Großmann, J., Grun, I., Mingram, J., Mrlina, J., Plessen, B., Stebich, M., Veress, C., Wendt, A., and Nowaczyk, N.: Reconnaissance study of an inferred Quaternary maar structure in the western part of the Bohemian Massif near Neualbenreuth, NE-Bavaria (Germany), Int. J. Earth Sci., 107, 1381–1405, https://doi.org/10.1007/s00531-017-1543-0, 2017.
Schimschal, S.: Seismic imaging of the crustal structure in the Münchberg/Vogtland/Erzgebirge area by reprocessing of the deep seismic reflection profile MVE90', MS thesis, TU Bergakademie, Freiberg, 2013.
Schreiber, U., Locker-Grütjen, O., and Mayer, C.: Hypothesis: origin of life in the deep-reaching tectonic faults, Orig. Life Evol. Biosph., 42, 47–54, https://doi.org/10.1007/s11084-012-9267-4, 2012.
Schuessler, J. A., Kämpf, H., Koch, U., and Alawi, M.: Earthquake impact on iron isotope signatures recorded in mineral spring water, J. Geophys. Res.-Sol. Ea., 121, 8548–8568, https://doi.org/10.1002/2016JB013408, 2016.
Tomek, Č., Dvořáková, V., and Vrána, S.: Geological interpretation of the 9HR and 503M seismic profiles in Western Bohemia, in: Geological Model of Western Bohemia Related to the KTB Borehole in Germany, edited by: Vrána, S. and Štedrá, V., J. Geol. Sci. Prague, 47, 43–50, 1997.
Umlauft, J. and Korn, M.: 3-D fluid channel location from noise tremors using matched field processing, Geophys. J. Int., 219, 1550–1561, https://doi.org/10.1093/gji/ggz385, 2019.
Vavryčuk, V.: Crustal anisotropy from local observations of shear-wave splitting in West Bohemia, Czech Republic, Bull. Seism. Soc. Am., 83, 1420–1441, 1993.
Vylita, T., Žák, K., Cílek, V., Hercman, H., and Mikšíková, L.: Evolution of hot-spring travertine accumulation in Karlovy Vary/Carlsbad (Czech Republic) and its significance for the evolution of Teplá valley and Ohře/Eger rift, Z. Geomorphol. N.F., 51, 427–442, 2007.
Wagner, G. A., Gögen, K., Jonckhere, R., Wagner, I., and Woda, C.: Dating of Quaternary volcanoes Komorní hůrka (Kammerbühl) and Železná hůrka (Eisenbühl), Czech Republic, by TL, ESR, alpha-recoil and fission track chronometry, Z. Geol. Wiss., 30, 191–200, 2002.
Weinlich, F. H., Bräuer, K., Kämpf, H., Strauch, G., Tesař, J., and Weise, S. M.: An active subcontinental mantle volatile system in the western Eger rift, Central Europe: Gas flux, isotopic (He, C, and N) and compositional fingerprints, Geochim. Cosmochim. Ac., 63, 3653–3671, 1999.
Weise, S. M., Bräuer, K., Kämpf, H., Strauch, G., and Koch, U.: Transport of mantle volatiles through the crust traced by seismically released fluids: A natural experiment in the earthquake swarm area Vogtland/NW Bohemia, central Europe, Tectonophysics, 336, 137–150, 2001.
Woith, H., Daskalopoulou, K., Zimmer, M., Fischer, T., Vlček, J., Trubač, J., Rosberg, J.-E., Vylita, T., and Dahm, T.: Multi-Level Gas Monitoring: A New Approach in Earthquake Research, Front. Earth Sci., 8, 585733, https://doi.org/10.3389/feart.2020.585733, 2020.
Wyss, M., Shimazaki, K., and Wiemer, S.: Mapping active magma chambers by b values beneath the off-Ito volcano, Japan, J. Geophys. Res., 102, 20413–20422, https://doi.org/10.1029/97JB01074, 1997.
- Abstract
- Main goals, overview processes and settings
- Current state, scientific aims and experimental approach
- Description of drillings, monitoring and scientific concepts
- Drilling and specific characteristics of individual drill holes
- Lithologies and well logging of boreholes
- Temperature measurements and the heat flow
- Seismic monitoring concept – the 3-D high-frequency seismic array at the S1 site
- Data management
- Summary
- Data availability
- Author contributions
- Competing interests
- Disclaimer
- Acknowledgements
- Financial support
- Review statement
- References
- Abstract
- Main goals, overview processes and settings
- Current state, scientific aims and experimental approach
- Description of drillings, monitoring and scientific concepts
- Drilling and specific characteristics of individual drill holes
- Lithologies and well logging of boreholes
- Temperature measurements and the heat flow
- Seismic monitoring concept – the 3-D high-frequency seismic array at the S1 site
- Data management
- Summary
- Data availability
- Author contributions
- Competing interests
- Disclaimer
- Acknowledgements
- Financial support
- Review statement
- References